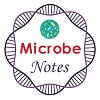
Microbe Notes

Extrachromosomal Inheritance: Types, Modes, Uses
Inheritance is the transfer of genetic information or traits from one cell or individual to another. While most inherited traits follow patterns of chromosomal inheritance, where genes on the chromosomes control the traits, there are some traits that do not follow this conventional pattern. These traits are caused by extrachromosomal inheritance.
Extrachromosomal inheritance, also known as cytoplasmic or extranuclear inheritance, refers to the inheritance of traits that are not controlled by chromosome genes. Instead, they are determined by genetic materials located outside the chromosomes . This form of inheritance occurs in the cytoplasm of cells and involves genes present in cytoplasmic organelles like mitochondria and plastids. The extrachromosomal hereditary factors have the ability to self-replicate and can be transmitted sexually or asexually. It is important to study these non-chromosomal factors to gain a comprehensive understanding of heredity.
The early recognition of extrachromosomal inheritance started with the demonstrations by Carl Correns, who observed that heredity is not solely governed by the nucleus. Correns demonstrated that hereditary factors can also be present in the cytoplasm, not just the nucleus. Over time, extrachromosomal inheritance was observed in many cases in plants and animals.
Table of Contents
Interesting Science Videos
Characteristics of Extrachromosomal Inheritance
There are several characteristics associated with extrachromosomal inheritance:
- Extrachromosomal inheritance does not follow the typical Mendelian inheritance patterns.
- The inheritance of extrachromosomal factors is independent of genes located within the cell nucleus.
- In some cases, extrachromosomal traits are inherited exclusively from the mother. This is because the egg contributes more cytoplasm to the zygote compared to the male parent.
- Extrachromosomal inheritance can lead to characteristic phenotypic changes that are not inherited in a Mendelian pattern.
- Extrachromosomal genes can exhibit vegetative (somatic) segregation which is rare in nuclear genes.
Types of Extrachromosomal Inheritance
There are two main types of extrachromosomal inheritance that are briefly discussed below:
1. Chloroplast inheritance
- Chloroplasts are organelles located in plant cells that play a vital role in photosynthesis. They possess their own DNA, known as chloroplast DNA (cpDNA), which is distinct from nuclear DNA.
- The inheritance of chloroplast genes was first discovered by Carl Correns and Erwin Baur in 1909.
- Correns conducted a study on Mirabilis jalapa , commonly known as the four o’clock plant, where he observed that the transmission of leaf color was strictly maternal, determined by the color of the ovule’s source.
- Baur found in his experiment in geranium ( Pelargonium zonale ) that chloroplast genes can also be inherited from both parents or from the male parent only, resulting in variegated plants.
- Recent research conducted at the Max Planck Institute of Molecular Plant Physiology with tobacco plants presents new evidence that challenges the commonly held belief that chloroplasts are solely inherited from the mother plant. The researchers discovered that under specific environmental conditions, chloroplasts from the father can also be passed on to the offspring.
2. Mitochondrial inheritance
- Mitochondria are cellular structures present in eukaryotic cells that are responsible for generating energy. They also contain their own unique DNA, known as mitochondrial DNA (mtDNA).
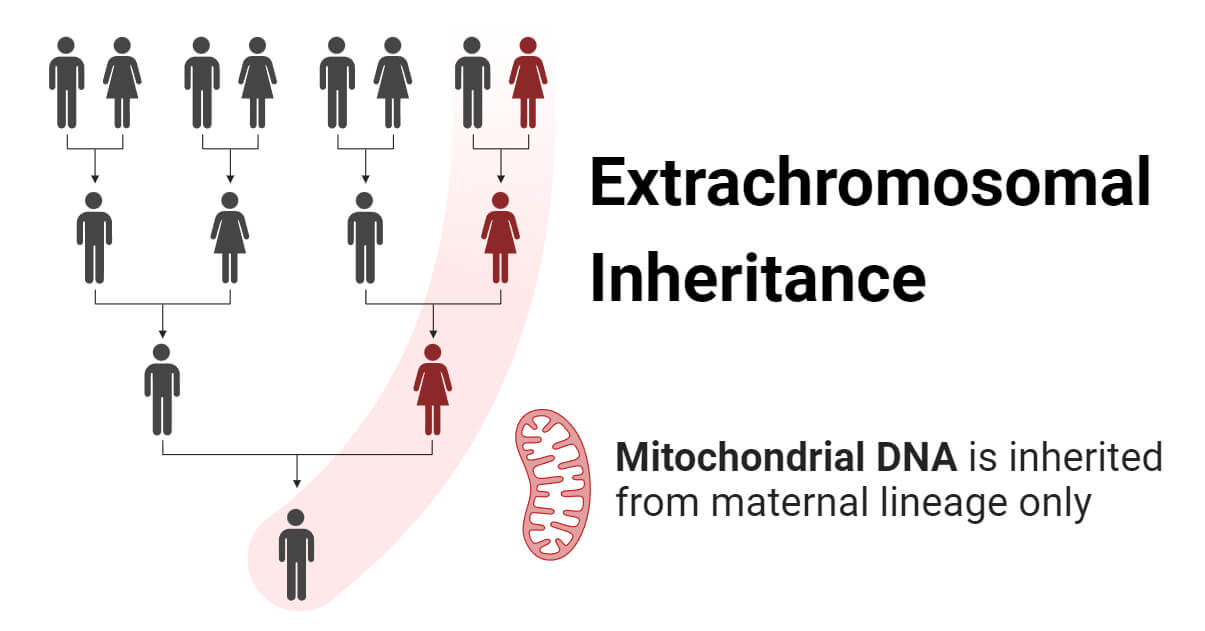
- mtDNA is the main form of extrachromosomal inheritance in animals. mtDNA is circular and encodes 37 genes on 16.5 kb of DNA.
- Margit and Sylvan Nass discovered the DNA in mitochondria in 1963.
- Mitochondria are primarily inherited uniparentally, mostly maternally. The zygote receives mitochondria exclusively from the mother, while the paternal contribution of mitochondria is minimal or negligible.
- In 2018, a controversial claim suggested that children can inherit mtDNA from their fathers. However, subsequent research found that in cases of biparental inheritance, mitochondrial DNA fragments can migrate into the nucleus and integrate with the chromosomes. These mitochondrial DNA fragments are inherited alongside the nuclear chromosomes but the primary inheritance of mitochondrial DNA still occurs from the mother. This research confirms that the concept of maternal inheritance is still true.
- MtDNA exhibits a higher rate of mutational change compared to nuclear DNA. Mutations in mtDNA can have significant effects and are associated with various diseases.
Modes of Extrachromosomal Inheritance
1. uniparental inheritance.
Uniparental inheritance refers to a mode of inheritance where genetic material or traits are inherited from a single parent, either the mother or the father. The genomes of extrachromosomal organelles are maternally inherited in most eukaryotes. For example, in humans, mitochondrial DNA is inherited solely from the mother. This is due to the significant contribution of cytoplasm from the egg to the zygote compared to the relatively minimal contribution from the sperm. Carl Correns’ experiments with four o’clock plants also demonstrated uniparental inheritance of chloroplast DNA, specifically through the maternal parent.
2. Biparental inheritance
Biparental inheritance is a less common form of extrachromosomal inheritance where genetic material from both parents contributes to the traits encoded by the extrachromosomal organelles. This can occur when there is a transfer of extrachromosomal genetic material from both the maternal and paternal parents to the offspring. Baur (1909) observed the inheritance of leaf phenotypes in Pelargonium cultivars, describing the transmission of chloroplasts through biparental inheritance.
3. Vegetative segregation
Vegetative segregation is a mode of extrachromosomal inheritance that involves the random distribution of cytoplasmic elements during cell division in asexual reproduction. In this process, extrachromosomal DNA within the cytoplasm is randomly segregated into daughter cells, resulting in unequal distribution of cytoplasmic content.
Differences between Extrachromosomal and Chromosomal Inheritance
Location | Genetic material is located in the cytoplasm or cytoplasmic organelles such as mitochondria or chloroplast. | Genetic material is located within the nucleus, specifically on chromosomes. |
Inheritance pattern | The inheritance patterns do not follow classic Mendelian principles. | Genetic traits on nuclear chromosomes generally follow Mendelian patterns of inheritance. |
Segregation pattern | Segregation is different from Mendelian segregation. Alleles may or may not segregate. | Chromosomes undergo precise and controlled segregation. |
Size | Extrachromosomal genetic elements are often smaller compared to nuclear chromosomes. | Nuclear chromosomes are larger and more complex structures. |
Parental inheritance | Extrachromosomal genes are often inherited from only one parent, known as uniparental inheritance. | Nuclear genes are inherited from both parents, following a biparental inheritance pattern. |
Significance of Extrachromosomal Inheritance
- Extrachromosomal inheritance is important for understanding evolutionary processes. It helps to study inheritance patterns and explore relationships between different species or groups.
- Maternal inheritance in extrachromosomal elements like mitochondrial DNA helps trace maternal lineages and study human population history. It is valuable for understanding ancestral relationships.
- Mutations or changes in extrachromosomal elements can cause genetic disorders or diseases. Studying the mechanisms of extrachromosomal inheritance is important for understanding these inherited disorders.
- Mitochondrial DNA has unique characteristics that make it useful for forensic identification. Its circular shape and multiple copies make it more resilient than nuclear DNA. The presence of specific genes and hypervariable regions enables mtDNA to act as a fingerprint for identification purposes.
- Extrachromosomal inheritance has also been useful in mapping the chloroplast and mitochondrial genomes in many species.
Challenges in studying Extrachromosomal Inheritance
- The lack of precise segregation in extrachromosomal inheritance makes it challenging to study the inheritance patterns of extrachromosomal genes.
- The complexity of extrachromosomal inheritance has led researchers to focus mainly on studying chromosomal factors, which are comparatively easier to understand.
- The exact characteristics and components involved in extrachromosomal inheritance are unclear, which makes it difficult to have a complete understanding of this process.
- Extrachromosomal hereditary factors could be lost through selection, whereas chromosomal inheritance tends to be more precise and regular.
- Birky, C. W. (1995). Uniparental inheritance of mitochondrial and chloroplast genes: mechanisms and evolution. Proceedings of the National Academy of Sciences, 92(25), 11331–11338. doi:10.1073/pnas.92.25.11331
- Birky, C. W. (2001). The Inheritance of Genes in Mitochondria and Chloroplasts: Laws, Mechanisms, and Models. Annual Review of Genetics, 35(1), 125–148. doi:10.1146/annurev.genet.35.102401.090231
- Birky, C. W. (2008). Uniparental inheritance of organelle genes. Current Biology, 18(16), R692–R695. doi:10.1016/j.cub.2008.06.049
- Camus, M. F., Sharbrough, J., & Hurst, G. D. (2022). Inheritance through the cytoplasm. Heredity, 129(1), 31-43. https://doi.org/10.1038/s41437-022-00540-2
- Chloroplast from the father | Max-Planck-Gesellschaft (mpg.de)
- Chung, K.P., Gonzalez-Duran, E., Ruf, S. et al. Control of plastid inheritance by environmental and genetic factors. Nat. Plants 9, 68–80 (2023). https://doi.org/10.1038/s41477-022-01323-7
- Cytoplasmic Inheritance: Meaning & Examples (unacademy.com)
- Esser, K., & Kuenen, R. (1967). Extrachromosomal inheritance. Genetics of Fungi, 439–468. doi:10.1007/978-3-642-86814-6_8
- Ferguson-Smith, A. C. (2001). Uniparental Inheritance. Brenner’s Encyclopedia of Genetics, 257–258. doi:10.1016/b978-0-12-374984-0.01605-3
- Gray, M. W. (2013). Mitochondrial DNA. Brenner’s Encyclopedia of Genetics, 436–438. doi:10.1016/b978-0-12-374984-0.00958-x
- https://www.mcgill.ca/oss/article/general-science/mitochondria-story-mothers-teenagers-and-energy
- Miko, I. (2008) Non-nuclear genes and their inheritance. Nature Education 1(1):135
- Pagnamenta, A. T., Wei, W., Rahman, S., & Chinnery, P. F. (2021). Biparental inheritance of mitochondrial DNA revisited. Nature Reviews Genetics, 22(8), 477–478. doi:10.1038/s41576-021-00380-6
- Weihe, A., Apitz, J., Pohlheim, F., Salinas-Hartwig, A., & Börner, T. (2009). Biparental inheritance of plastidial and mitochondrial DNA and hybrid variegation in Pelargonium. Molecular Genetics and Genomics, 282(6), 587-593. https://doi.org/10.1007/s00438-009-0488-9
About Author
Sanju Tamang
Leave a Comment Cancel reply
Save my name, email, and website in this browser for the next time I comment.
This site uses Akismet to reduce spam. Learn how your comment data is processed .

for World Environment Day with code NATURE30
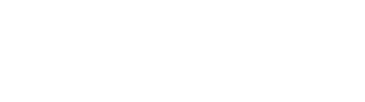
Share this article

Table of Contents
Latest updates.
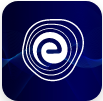
1 Million Means: 1 Million in Rupees, Lakhs and Crores
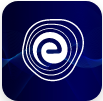
Ways To Improve Learning Outcomes: Learn Tips & Tricks
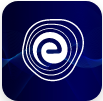
Visual Learning Style for Students: Pros and Cons
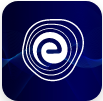
NCERT Books for Class 6 Social Science 2024 – Download PDF
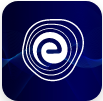
CBSE Syllabus for Class 9 Social Science 2023-24: Download PDF
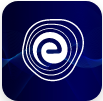
CBSE Syllabus for Class 8 Maths 2024: Download PDF
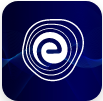
NCERT Books for Class 6 Maths 2025: Download Latest PDF
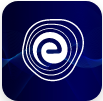
CBSE Class 10 Study Timetable 2024 – Best Preparation Strategy
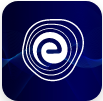
CBSE Class 10 Syllabus 2025 – Download PDF
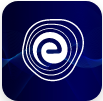
CBSE Syllabus for Class 11 2025: Download PDF
Tag cloud :.
- entrance exams
- engineering
- ssc cgl 2024
- Written By Jyotirmayee Nayak
- Last Modified 21-06-2023
Extrachromosomal Inheritance: Definition, Criteria & Examples
Extrachromosomal Inheritance: Do you know that our genetic material, i.e., DNA, is arranged on chromosomes that store, transfer, and express the genetic information? Nuclear DNA that is present in the nucleus of a cell controls all the phenotypes that are transferred from the parents to offspring, and this process is called Mendelian Inheritance.
DNA is also present in mitochondria and plastids. Before fertilization, a sperm cell loses most of its cytoplasm and cytoplasmic organelles, and only the sperm nucleus enters the egg. So, the zygote receives the genome of plastids and mitochondria only from the maternal parent, and thus this phenomenon is known as extra chromosomal inheritance or cytoplasmic inheritance. Read this article to know more about extrachromosomal inheritance , how it occurs, and some non-mendelian inheritance examples.
What is Extrachromosomal Inheritance?
Extrachromosomal Inheritance is defined as a form of a non-mendelian inheritance that is governed by the DNA present in the cytoplasm. It refers to the transmission of genes that occurs outside the nucleus, also known as extranuclear inheritance, found in most eukaryotes.
It commonly occurs in the cytoplasmic organelles such as mitochondria and plastids or in cellular parasites like viruses or bacteria. The traits present in the mitochondria or plastids do not follow the Mendelian principles of inheritance. It was first reported by Carl Correns in 1908 in Mirabilis jalapa (Four o’clock plant) plastid DNA for leaf colour. The extrachromosomal genome consists of a few genes of several thousand base pairs, mostly encoding rRNA, tRNA, and other proteins for their DNA metabolism.
No cytoplasm is contributed by the male gamete, which means whatever cytoplasm is present in the zygote is contributed by the female gamete only. Since this cytoplasmic inheritance is coming from the mother or maternal side, this inheritance is also referred to as maternal or uniparental inheritance.

Fig: Diagrammatic Representation of Cytoplasmic Inheritance
Criteria for Extrachromosomal Inheritance
The criteria for extrachromosomal inheritance are as follows: 1. The extrachromosomal DNA does not follow the Mendelian pattern of inheritance, unlike the common nuclear DNA. 2. The extrachromosomal DNA doesn’t have its own protein synthetic machinery for the process of replication, transcription, and translation. So, it synthesizes its own DNA and makes its own protein. 3. The extrachromosomal DNA is inherited from the maternal side because the female gamete contains more cytoplasm than the male gamete. 4. All the progenies obtained by this inheritance have the phenotype of only one parent, i.e., the mother. 5. The extranuclear genes present in the mitochondria and plastids cannot be mapped to the chromosomes in the nucleus. 6. This inheritance is not affected by substituting the nucleus with a different genotype.
Extrachromosomal Inheritance Examples
There is no scientific evidence for the occurrence of extrachromosomal inheritance. However, according to one theory, the female gamete (egg/ovum) is bigger than the male gamete (sperm), which contains more cytoplasm and more organelles. This influences the extrachromosomal inheritance or maternal inheritance.
Following are some examples of extrachromosomal inheritance:
1. Plastid Inheritance in Mirabilis jalapa (4 o’clock plant) (a) Correns, in 1908 worked out on the plastid inheritance in Mirabilis jalapa, which shows two types of branching, i.e., branches with dark green leaves and branches with pale green leaves.

Fig: Leaf Variegation in Mirabilis jalapa
(b) When a dark green leaved plant (male) is crossed with a pale green leaved plant (female), all the F 1 offspring obtained have pale green leaves only, which shows that the inheritance of plastid is a maternal inheritance.
(c) Similarly, when a pale green leaved plant (male) is crossed with a dark green leaved plant (female), all the F 1 offspring obtained have dark green leaves only, which shows that the inheritance of plastid is a maternal inheritance.
(d) From the above cross, it is clear that this inheritance does not involve nuclear genes. It is due to the chloroplast gene found in the ovum of the female plant, which provides the cytoplasm during fertilization as the male gamete contributes only to the nucleus but no cytoplasm.

Fig: Plastid Inheritance in Mirabilis jalapa
2. Cytoplasmic male sterility in maize (a) The male sterility in maize is inherited through the cytoplasm of the egg in offspring. (b) When we cross a male sterile plant with a normal fertile plant, all the F 1 plants produced are sterile. (c) Now, when all the F 1 sterile plants are backcrossed with a normal fertile plant, then all the F 2 plants produced are also sterile.
3. The maternal effect in snail (a) The coiling of snails is governed by maternal inheritance. (b) There are two types of snail coiling phenotypes: (i) Dextral (coiling for right side) (ii) Sinistral (coiling for left side) (c) In the development of coiling, the mother’s genotype is exclusively responsible.
Thus, extrachromosomal or extranuclear inheritance or cytoplasmic inheritance is a form of non-mendelian inheritance, which is defined as the transmission of genes outside the nucleus that is found in most eukaryotes. It commonly occurs in the cytoplasmic organelles such as mitochondria and chloroplasts or in cellular parasites like viruses or bacteria. Extrachromosomal inheritance is also known as maternal inheritance because this type of inheritance is governed by the maternal genes and not the nuclear genes. This inheritance does not follow the Mendelian pattern of inheritance.
Q.1: What is extrachromosomal inheritance? Ans: Extrachromosomal Inheritance is defined as a form of a non-mendelian pattern of inheritance that is governed by the DNA present in the cytoplasm, which refers to the transmission of genes outside the nucleus.
Q.2: What are the criteria for extrachromosomal inheritance? Ans: The criteria for extrachromosomal inheritance are as follows: (a) The extrachromosomal DNA does not follow the Mendelian pattern of inheritance, unlike the common nuclear DNA. (b) The extrachromosomal DNA doesn’t have its own protein synthetic machinery for the process of replication, transcription, and translation. So, it synthesizes its own DNA and makes its own protein. (c) The extrachromosomal DNA is inherited from the maternal side because the female gamete contains more cytoplasm than the male gamete.
Q.3: Who first reported the extrachromosomal inheritance? Ans: Carl Correns, in 1908, first reported about the extrachromosomal inheritance in Mirabilis jalapa (Four o’clock plant) plastid DNA for leaf colour.
Q.4: What are the examples of extrachromosomal inheritance? Ans: The examples of extrachromosomal inheritance are as follows: 1. Cytoplasmic male sterility in maize 2. Maternal effect in snail 3. Inheritance of kappa particles in Paramecium
Q.5. Which parent contributes more to cytoplasmic inheritance? Ans : The female parent contributes more to cytoplasmic inheritance.
Related Articles
1 Million Means: 1 million in numerical is represented as 10,00,000. The Indian equivalent of a million is ten lakh rupees. It is not a...
Ways To Improve Learning Outcomes: With the development of technology, students may now rely on strategies to enhance learning outcomes. No matter how knowledgeable a...
Visual Learning Style: We as humans possess the power to remember those which we have caught visually in our memory and that too for a...
NCERT Books for Class 6 Social Science 2024: Many state education boards, including the CBSE, prescribe the NCRET curriculum for classes 1 to 12. Thus,...
CBSE Syllabus for Class 9 Social Science: The Central Board of Secondary Education releases the revised CBSE Class 9 Social Science syllabus. The syllabus is...
CBSE Syllabus for Class 8 Maths 2023-24: Students in CBSE Class 8 need to be thorough with their syllabus so that they can prepare for the...
NCERT Books for Class 6 Maths 2025: The National Council of Educational Research and Training (NCERT) textbooks are the prescribed set of books for schools...
CBSE Class 10 Study Timetable: The CBSE Class 10 is the board-level exam, and the Class 10th students will appear for the board examinations for...
CBSE Class 10 Syllabus 2025: The Central Board of Secondary Education (CBSE) conducts the Class 10 exams every year. Students in the CBSE 10th Class...
CBSE Syllabus for Class 11 2025: The Central Board of Secondary Education (CBSE) has published the Class 11 syllabus for all streams on its official...
NCERT Solutions for Class 7 Science Chapter 16 Water – A Precious Resource
NCERT Solutions for Class 7 Science Chapter 16 Water – A Precious Resource: In this chapter, students will study about the importance of water. There are three...
NCERT Solutions for Class 7 Science Chapter 10 2024: Respiration in Organisms
NCERT Solutions for Class 7 Science Chapter 10 Respiration in Organisms: NCERT solutions are great study resources that help students solve all the questions associated...
Factors Affecting Respiration: Definition, Diagrams with Examples
In plants, respiration can be regarded as the reversal of the photosynthetic process. Like photosynthesis, respiration involves gas exchange with the environment. Unlike photosynthesis, respiration...
NCERT Solutions for Class 7 Science Chapter 12
NCERT Solutions for Class 7 Science Chapter 12 Reproduction in Plants: The chapter 'Reproduction' in Class 7 Science discusses the different modes of reproduction in...
NCERT Solutions for Class 7 Science Chapter 11
NCERT Solutions for Class 7 Science Chapter 11: Chapter 11 of Class 7 Science deals with Transportation in Animals and Plants. Students need to ensure...
NCERT Solutions for Class 7 Science Chapter 15: Light
NCERT Solutions for Class 7 Science Chapter 15: The NCERT Class 7 Science Chapter 15 is Light. It is one of the most basic concepts. Students...
NCERT Solutions for Class 7 Science Chapter 13
NCERT Solutions for Class 7 Science Chapter 13: Chapter 13 in class 7 Science is Motion and Time. The chapter concepts have a profound impact...
NCERT Solutions for Class 7 Science Chapter 14: Electric Current and its Effects
NCERT Solutions for Class 7 Science Chapter 14: One of the most important chapters in CBSE Class 7 is Electric Current and its Effects. Using...
General Terms Related to Spherical Mirrors
General terms related to spherical mirrors: A mirror with the shape of a portion cut out of a spherical surface or substance is known as a...
Animal Cell: Definition, Diagram, Types of Animal Cells
Animal Cell: An animal cell is a eukaryotic cell with membrane-bound cell organelles without a cell wall. We all know that the cell is the fundamental...
NCERT Solutions for Class 10 Science 2024 – Download PDF
NCERT Solutions for Class 10 Science: The National Council of Educational Research and Training (NCERT) publishes NCERT Solutions for Class 10 Science as a comprehensive...
NCERT Books for Class 12 Chemistry 2024: Download PDF
NCERT Books for class 12 Chemistry: NCERT publishes chemistry class 12 books every year. The NCERT chemistry class 12 books are essential study material for...
CBSE Class 9 Mock Tests 2025: Attempt Online Mock Test Series (Subject-wise)
We all have heard at least once that the secret to success is practice. Some of you could say it's a cliché, but those who...
NCERT Books for Class 10 Maths 2025: Download Latest PDF
NCERT Books for Class 10 Maths: The NCERT Class 10 Maths Book is a comprehensive study resource for students preparing for their Class 10 board exams....
Arc of a Circle: Definition, Properties, and Examples
Arc of a circle: A circle is the set of all points in the plane that are a fixed distance called the radius from a fixed point...
CBSE Class 10 Mock Test 2025: Practice Latest Test Series
CBSE Class 10 Mock Test 2025: Students' stress is real due to the mounting pressure of scoring good marks and getting into a renowned college....
NCERT Solutions for Class 10 2024: Science and Maths
NCERT Solutions for Class 10 2024: Students appearing for the CBSE Class 10 board exam must go through NCERT Solutions to prepare for the exams...
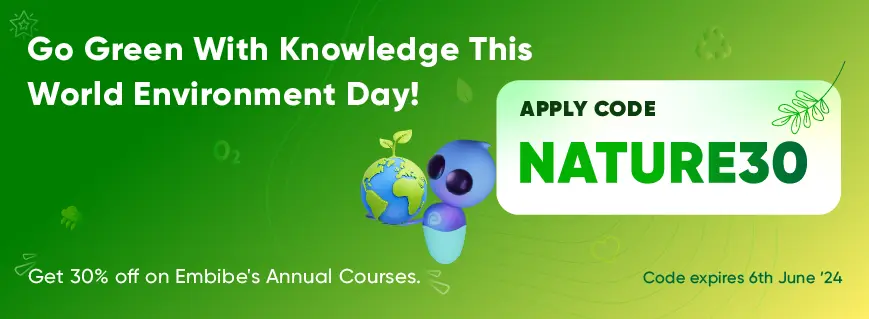
39 Insightful Publications
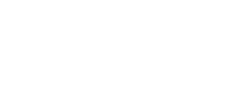
Embibe Is A Global Innovator
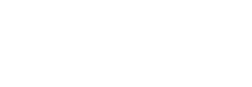
Innovator Of The Year Education Forever
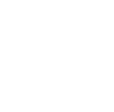
Interpretable And Explainable AI
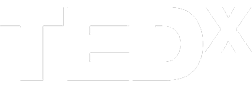
Revolutionizing Education Forever
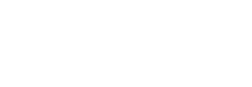
Best AI Platform For Education
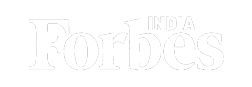
Enabling Teachers Everywhere
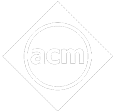
Decoding Performance
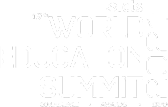
Leading AI Powered Learning Solution Provider
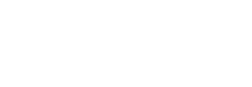
Auto Generation Of Tests
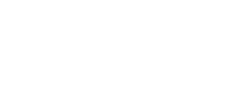
Disrupting Education In India
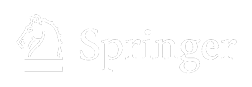
Problem Sequencing Using DKT
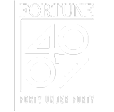
Help Students Ace India's Toughest Exams
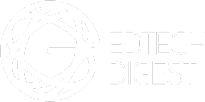
Best Education AI Platform
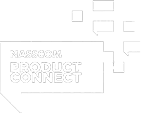
Unlocking AI Through Saas
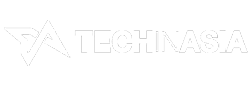
Fixing Student’s Behaviour With Data Analytics
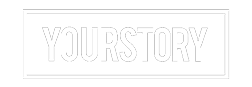
Leveraging Intelligence To Deliver Results
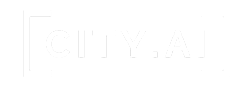
Brave New World Of Applied AI
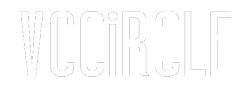
You Can Score Higher
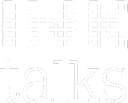
Harnessing AI In Education
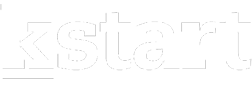
Personalized Ed-tech With AI
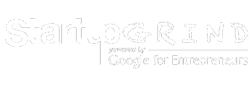
Exciting AI Platform, Personalizing Education
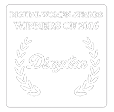
Disruptor Award For Maximum Business Impact
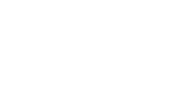
Top 20 AI Influencers In India
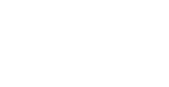
Proud Owner Of 9 Patents
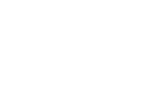
Innovation in AR/VR/MR

Best Animated Frames Award 2024

Trending Searches
Previous year question papers, sample papers.
Unleash Your True Potential With Personalised Learning on EMBIBE
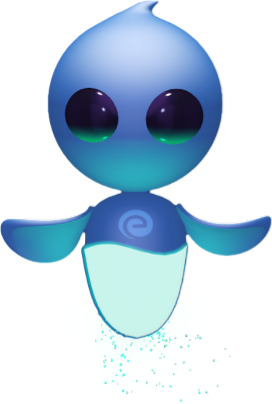
Ace Your Exam With Personalised Learning on EMBIBE
Enter mobile number.
By signing up, you agree to our Privacy Policy and Terms & Conditions
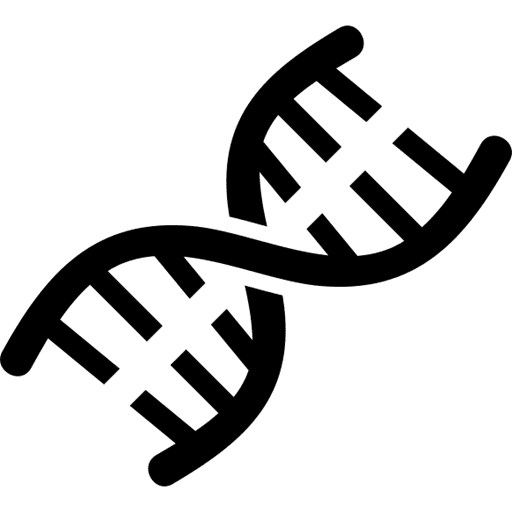
Understanding the Fascinating World of Extra Chromosomal Inheritance
- Post author By admin-science
- Post date 20.12.2023
Chromosomal inheritance is the process by which genetic information is passed down from parent to offspring through the transmission of chromosomes. These chromosomes contain the genes that determine various traits and characteristics in organisms. The study of chromosomal inheritance has helped scientists uncover the complex mechanisms by which genes are inherited and expressed.
While the majority of inheritance patterns follow the principles of Mendelian genetics, there are cases where genetic material is transmitted through other mechanisms. One such mechanism is extra chromosomal inheritance , which involves the transmission of genetic information through non-chromosomal structures, such as mitochondria.
Mitochondria are small organelles found in cells that play a crucial role in energy production. They contain their own DNA, known as mitochondrial DNA (mtDNA), which is inherited maternally. Unlike chromosomal genes, which are inherited from both parents, mtDNA is only inherited from the mother. This unique mode of transmission has important implications for understanding diseases and phenotypic traits that are influenced by mitochondrial mutations.
Studying extra chromosomal inheritance has provided valuable insights into the impact of mitochondrial mutations on an individual’s phenotype. Mutations in mtDNA have been linked to a range of disorders, including mitochondrial diseases, neurodegenerative disorders, and age-related conditions. Understanding the mechanisms behind extra chromosomal inheritance can lead to better diagnostic tools and potential therapies for these conditions.
What is Extra Chromosomal Inheritance?
Extra chromosomal inheritance refers to the transmission of genetic information through cellular structures other than the chromosomes found in the cell nucleus. This type of inheritance occurs outside of the typical Mendelian patterns that govern chromosomal inheritance. One of the most well-known examples of extra chromosomal inheritance involves the mitochondria.
Mitochondria and Extra Chromosomal Inheritance:
Mitochondria are double-membraned organelles found in the cells of eukaryotic organisms. They are responsible for producing energy in the form of adenosine triphosphate (ATP). While most of an organism’s genetic information is stored in the nuclear genome, mitochondria have their own small circular DNA molecules called mitochondrial DNA (mtDNA).
Mitochondrial inheritance follows a unique pattern as mtDNA is passed down from generation to generation. Unlike chromosomal inheritance, which involves a combination of DNA from both parents, mitochondrial DNA is inherited exclusively from the mother. This means that all offspring inherit their mtDNA only from their mother, regardless of the father’s genetic contribution.
Genes, Phenotype, and Extra Chromosomal Inheritance:
The genes contained in mitochondrial DNA play a crucial role in various cellular functions, particularly those related to energy production. Mutations in these genes can lead to mitochondrial disorders, which can affect different organ systems in the body.
Extra chromosomal inheritance through mitochondria can impact the phenotype, or the observable characteristics of an organism. Since mitochondria are responsible for energy production, mutations in mitochondrial DNA can lead to impaired energy production in cells. This can result in a range of symptoms and disorders, including muscle weakness, developmental delays, and neurological problems.
In summary, extra chromosomal inheritance refers to the transmission of genetic information through structures other than the chromosomes located in the cell nucleus. Mitochondria are a prime example of extra chromosomal inheritance, with their own unique mode of inheritance through mitochondrial DNA. Mutations in mitochondrial DNA can have significant implications for an organism’s phenotype and can result in various disorders.
The Role of Mitochondrial DNA
Mitochondrial DNA (mtDNA) plays a vital role in extra chromosomal inheritance. Unlike nuclear DNA, which is inherited from both parents, mtDNA is exclusively inherited from the mother.
The mitochondria, often referred to as the “powerhouses” of the cell, are responsible for energy production through oxidative phosphorylation. They have their own set of genes, separate from the nuclear genome, that are essential for mitochondrial function.
Since offspring primarily inherit their mtDNA from their mother, any mutations in the mitochondrial genes can lead to changes in phenotypes. These changes can manifest as various diseases and disorders, as the affected genes play a crucial role in cellular metabolism and energy production.
Furthermore, the transmission of mitochondrial mutations follows a unique pattern. While nuclear DNA undergoes recombination during gamete formation, mtDNA is usually transmitted intact from one generation to the next.
Due to the higher mutation rate and lack of recombination, mtDNA mutations can accumulate over time. This can result in a phenomenon known as heteroplasmy, where different copies of mtDNA with varying mutations coexist within an individual. The levels of heteroplasmy can influence the severity and progression of inherited mitochondrial diseases.
In conclusion, mitochondrial DNA and its inheritance have significant implications for the understanding of extra chromosomal inheritance and the development of genetic disorders. By studying the role of mtDNA and its mutations, researchers can gain insights into various aspects of inheritance, phenotype expression, and disease progression.
Inheritance of Genes through Plasmids
When bacteria divide, plasmids can be replicated and passed on to the daughter cells along with the chromosomal DNA. This means that the genes carried by the plasmids can also be inherited by subsequent generations of bacteria. This mode of inheritance is known as extra chromosomal inheritance.
Unlike chromosomal inheritance, the transmission of genes through plasmids is not subject to the same strict rules of Mendelian genetics. Plasmids can be transferred between different bacterial species through horizontal gene transfer, allowing for the spread of useful genes across a wide range of organisms.
The inheritance of genes through plasmids can have significant implications for the phenotype of the organisms involved. For example, the transmission of antibiotic resistance genes through plasmids can lead to the emergence of drug-resistant strains of bacteria, posing a significant threat to human health.
Furthermore, mutations in plasmid genes can also occur, leading to changes in the traits encoded by those genes. This can result in the rapid evolution of new traits and adaptations in response to changing environmental conditions.
Overall, the understanding of the inheritance of genes through plasmids provides valuable insights into the mechanisms of genetic variation and adaptation. It highlights the dynamic nature of genetic information and its potential for shaping the phenotypic diversity observed in the natural world.
Understanding the Mechanisms of Epigenetic Inheritance
Epigenetic inheritance refers to the transmission of information from one generation to the next through modifications in gene expression patterns, rather than changes in the DNA sequence itself. These modifications can alter the activity of genes and can be influenced by a variety of factors, including environmental cues and lifestyle choices.
Exploring Epigenetic Mutations
One of the key mechanisms of epigenetic inheritance is the presence of extra chromosomal DNA, such as mitochondrial DNA, in the cells of offspring. Mitochondria are small organelles responsible for producing energy in the cell. They contain their own set of genes, separate from the genes found in the cell’s nucleus.
Epigenetic mutations in mitochondrial DNA can result in a wide range of effects on the phenotype of the offspring. These mutations can alter the functioning of the mitochondria, leading to a variety of health conditions, including mitochondrial diseases. The severity of these conditions can vary depending on the specific mutations and the extent of their effects on the mitochondria.
The Role of Epigenetic Inheritance in Gene Regulation
Epigenetic inheritance also plays a crucial role in gene regulation. It can influence the activity of certain genes, turning them on or off, and thereby impacting the development and functioning of an organism. This can have significant implications for the phenotypic traits that are passed on from one generation to the next.
Epigenetic modifications can work in conjunction with genetic mutations to shape an individual’s phenotype. For example, a genetic mutation may result in the production of a defective protein, but epigenetic modifications can help regulate the expression of that gene and mitigate the impact of the mutation.
A better understanding of the mechanisms of epigenetic inheritance is essential for unraveling the complexities of inheritance patterns and their role in shaping the phenotype of an organism. This knowledge can have important implications for fields such as genetics, medicine, and evolutionary biology.
Key Concepts | Perspectives |
---|---|
Extra chromosomal DNA | Environmental cues |
Mitochondrial DNA | Lifestyle choices |
Epigenetic mutations | Mitochondrial diseases |
Gene regulation | Genetic mutations |
Phenotype | Evolutionary biology |
Exploring the Role of Extrachromosomal Circular DNAs in Inheritance
Understanding the mechanisms of inheritance is essential for unraveling the mysteries of genetics and evolution. While chromosomal DNA is traditionally considered the primary carrier of genetic information, recent research has shed light on the role of extrachromosomal circular DNAs (eccDNAs) in inheritance.
Mutations in chromosomal DNA can lead to changes in phenotype, but it is becoming increasingly clear that eccDNAs play a crucial role in shaping the genetic landscape. These circular DNAs can arise from various sources, including mitochondria, and carry unique sets of genes that can influence inheritance patterns.
EccDNAs can be passed on to offspring through both maternal and paternal lineages, allowing for the transmission of specific genes or mutations. This mode of inheritance adds an extra layer of complexity to genetic studies, as it can contribute to heterogeneity within populations.
One of the most fascinating aspects of eccDNAs is their potential impact on gene expression. While chromosomal DNA is tightly regulated, eccDNAs can escape the traditional mechanisms of gene silencing and gain autonomy. This autonomous behavior can result in altered gene expression profiles and phenotypic variations not solely determined by the chromosomal genotype.
Studies have shown that eccDNAs can interact with chromosomal DNA, influencing gene regulation and potentially leading to phenotypic diversity. The dynamic nature of eccDNAs allows for rapid adaptation to changing environmental conditions, providing a selective advantage for organisms carrying them.
Additionally, eccDNAs can undergo replication and segregation independent of chromosomal DNA, further contributing to their inheritance potential. This unique feature allows for the enrichment or depletion of specific genes or mutations in populations over time.
As the scientific community delves deeper into the world of extrachromosomal circular DNAs, many questions remain unanswered. Further research is needed to fully understand the mechanisms underlying eccDNA inheritance and its implications for genetic diversity and evolution.
In conclusion, the role of extrachromosomal circular DNAs in inheritance is an emerging field of study with significant implications for our understanding of genetics. By exploring the unique properties of eccDNAs and their interactions with chromosomal DNA, we can gain insights into the complex processes that shape the phenotypic diversity of organisms.
Advantages and Disadvantages of Extra Chromosomal Inheritance
Extra chromosomal inheritance refers to the transmission of genetic material through the mitochondria, which are organelles found in eukaryotic cells. Unlike chromosomal inheritance, which involves the transmission of genes through the chromosomes, extra chromosomal inheritance has unique advantages and disadvantages.
Advantages :
- Maternal Inheritance: One of the advantages of extra chromosomal inheritance is that it is exclusively passed down from the mother to her offspring. This means that the mitochondrial DNA is not subject to recombination and offspring inherit it solely from their mother.
- Phenotype Control: The presence of genes in the mitochondria allows for the direct control of certain phenotypic traits. This is because mitochondria play a crucial role in cellular energy production and metabolism, and variations in mitochondrial genes can directly impact these processes.
- Rapid Evolution: Extra chromosomal inheritance has the potential to facilitate rapid evolutionary changes. The small size and high mutation rate of mitochondrial DNA can lead to the accumulation of genetic variations more quickly compared to chromosomal DNA.
Disadvantages :
- Limited Genetic Diversity: Due to the maternal inheritance pattern, extra chromosomal inheritance can result in limited genetic diversity. Offspring receive the same set of mitochondrial genes from their mother, which reduces the potential for genetic variation.
- Prone to Mutations: The high mutation rate of mitochondrial DNA can lead to the accumulation of harmful mutations. This can result in mitochondrial disorders and diseases, as well as an increased risk of certain health conditions in offspring.
- Incomplete Penetrance: In some cases, the expression of mitochondrial genes may not always result in a visible phenotype. This phenomenon, called incomplete penetrance, can complicate the study and understanding of extra chromosomal inheritance.
Overall, extra chromosomal inheritance offers unique advantages and disadvantages compared to chromosomal inheritance. It plays a significant role in shaping the phenotype of organisms, but also poses challenges in terms of genetic diversity and the potential for harmful mutations.
Potential Benefits of Extra Chromosomal Inheritance
Extra chromosomal inheritance is an alternative form of inheritance that involves the transmission of genetic material independent of the chromosomal DNA. While this mechanism is relatively rare compared to traditional chromosomal inheritance, it offers several potential benefits.
One potential benefit is the ability to transfer specific traits or genes to offspring without the risk of dilution or recombination. With extra chromosomal inheritance, genes can be passed down intact from one generation to the next, ensuring that the desired phenotype is maintained. This can be particularly advantageous when it comes to preserving unique or advantageous traits in a population.
Another potential benefit is the potential for rapid genetic evolution. Extra chromosomal inheritance allows for the transmission of genetic material that can bypass the slow process of chromosomal mutations. This means that new genetic variations can be quickly introduced and spread throughout a population, leading to potentially faster adaptation to changing environments or selective pressures.
Furthermore, extra chromosomal inheritance can also provide a mechanism for the transmission of genetic material that is independent of chromosomal mechanisms. This can be especially valuable in cases where chromosomal abnormalities or mutations prevent the normal transmission of genetic information. The extra chromosomal transmission can help to mitigate the impact of such mutations and ensure the survival of certain genes or traits.
In conclusion, while extra chromosomal inheritance may be relatively rare, it offers several potential benefits. These include the ability to maintain specific traits or genes across generations, the potential for rapid genetic evolution, and the ability to bypass chromosomal abnormalities or mutations. Further research is needed to fully understand the mechanisms and implications of extra chromosomal inheritance, but it undoubtedly plays a valuable role in genetic diversity and adaptation.
Potential Drawbacks of Extra Chromosomal Inheritance
Extra chromosomal inheritance refers to the transmission of genetic information through non-nuclear genetic elements, such as mitochondria, in addition to the traditional nuclear genes. While this mode of inheritance offers several advantages, it also presents potential drawbacks that should be considered.
1. Limited Genetic Variation
One potential drawback of extra chromosomal inheritance is the limited genetic variation that can be passed on to offspring. Unlike nuclear genes, which undergo recombination and can generate new combinations of alleles, extra chromosomal genes do not undergo this process. As a result, the genetic variation in extra chromosomal inheritance is restricted to the existing pool of genes present in the mitochondria or other non-nuclear genetic elements. This limited genetic variation can hinder the ability of populations to adapt to changing environments and may increase the risk of genetic diseases.
2. Impact on Phenotype
Extra chromosomal inheritance can also have an impact on the phenotype of offspring due to the close relationship between genes and mitochondria. Mitochondria play a crucial role in cellular energy production, and mutations in the mitochondrial genome can lead to a variety of disorders, including neurological and metabolic conditions. Therefore, if an individual inherits a mitochondrial mutation through extra chromosomal inheritance, it can affect their health and overall phenotype. This dependence on mitochondria for both energy production and genetic material transmission can make extra chromosomal inheritance more vulnerable to the effects of harmful mutations.
While extra chromosomal inheritance offers unique insights into the complex nature of genetic inheritance, it is important to recognize and consider the potential drawbacks associated with this mode of transmission. Understanding these limitations can help researchers and scientists develop strategies to mitigate the negative impacts and maximize the benefits of extra chromosomal inheritance in various fields of study.
Study of Extra Chromosomal Inheritance in Model Organisms
The study of extra chromosomal inheritance is crucial for understanding the transmission of genetic information that is not carried by the conventional nuclear chromosomal DNA. Extra chromosomal inheritance refers to the transmission of genetic material through mechanisms other than the regular Mendelian inheritance of alleles through chromosomes.
Model organisms have played a significant role in unraveling the complexities of extra chromosomal inheritance. These organisms, such as yeast, flies, and worms, have provided valuable insights into the various processes and mechanisms involved in this type of inheritance.
One prominent example of extra chromosomal inheritance is observed in mitochondria. Mitochondria possess their own separate genome, known as mitochondrial DNA (mtDNA). The transmission of mtDNA occurs predominantly through maternal inheritance, where offspring inherit their mtDNA exclusively from their mother.
This mode of inheritance has important implications for the phenotype of offspring. Mutations that occur in mtDNA can result in mitochondrial diseases and genetic disorders. Understanding how these mutations are transmitted and their effects on the phenotype of the offspring is essential for diagnosing and treating such conditions.
Model organisms provide a platform for studying the molecular mechanisms underlying extra chromosomal inheritance in a controlled laboratory setting. They allow researchers to manipulate and study various aspects of this phenomenon, including the transmission of non-chromosomal genetic information and the inheritance patterns of extra chromosomal elements.
Through the study of model organisms, scientists have been able to uncover the intricate interactions between nuclear chromosomal DNA and extra chromosomal elements, shedding light on the complexities of inheritance beyond the conventional Mendelian principles.
In conclusion, the study of extra chromosomal inheritance in model organisms has provided invaluable insights into the transmission of genetic material through mechanisms other than conventional chromosomal inheritance. This research has helped to unravel the complexities of inheritance patterns involving extra chromosomal elements, such as mitochondria, and has enhanced our understanding of the molecular mechanisms underlying these processes.
Research on Extra Chromosomal Inheritance in Yeast
Yeast is a popular model organism for studying various biological phenomena, including extra chromosomal inheritance. Extra chromosomal inheritance refers to the transmission of genetic information through elements outside of the chromosomal genome.
In yeast, one of the most well-known examples of extra chromosomal inheritance is the inheritance of mitochondrial DNA (mtDNA). Mitochondria are organelles found in eukaryotic cells that are responsible for energy production. They have their own small circular genome, separate from the nuclear genome.
Research on extra chromosomal inheritance in yeast has revealed important insights into the transmission and stability of mtDNA. Mutations in genes involved in mtDNA replication, repair, and segregation can lead to changes in the phenotype of the yeast offspring.
One key finding in yeast research is the phenomenon of petite mutants. These mutants have lost a significant portion of their mtDNA and exhibit reduced mitochondrial function. The petite phenotype can be inherited from the mother cell to the daughter cells, indicating the presence of extra chromosomal mechanisms for transmitting mtDNA.
Studies on extra chromosomal inheritance in yeast have also shed light on the role of nuclear genes in regulating mtDNA stability. Mutations in nuclear genes can affect the replication and maintenance of mtDNA, leading to changes in the phenotype of the yeast offspring.
The study of extra chromosomal inheritance in yeast has not only deepened our understanding of the mechanisms of mtDNA transmission but has also provided important insights into the broader field of extra chromosomal inheritance. Further research in this field will continue to uncover new and exciting discoveries.
Key Concepts | Perspectives |
---|---|
Extra chromosomal inheritance | Exploring the mechanisms of transmission |
Mitochondrial DNA | Investigating its structure and function |
Mutations | Understanding their effects on phenotype |
Genes | Identifying their role in inheritance |
Phenotype | Linking genotype to observable traits |
Inheritance | Unraveling the patterns of genetic transfer |
Offspring | Investigating the characteristics of descendant cells |
Studying Extra Chromosomal Inheritance in Bacteria
Extra chromosomal inheritance refers to the transmission of genetic material that is not located on the chromosomal DNA. This phenomenon has been extensively studied in bacteria, where it has provided valuable insights into the understanding of inheritance and the resulting phenotypes in offspring.
One of the key examples of extra chromosomal inheritance in bacteria is the transmission of genetic material through plasmids. Plasmids are small, circular DNA molecules that can replicate independently of the chromosomal DNA. They can carry a wide range of genes that can confer various traits to the bacterial cell and its offspring. The transfer of plasmids can occur through conjugation, where a donor bacterium directly transfers the plasmid to a recipient bacterium.
The transmission of plasmids through conjugation can lead to the inheritance of specific phenotypes in the offspring. This is because the plasmids often carry genes that provide the bacterium with advantages, such as antibiotic resistance. When the plasmid is transferred to the offspring, they also inherit the resistance to antibiotics.
Another example of extra chromosomal inheritance in bacteria is the transmission of genetic material through bacteriophages. Bacteriophages are viruses that infect bacteria and can integrate their genetic material into the bacterial chromosome. This integration can result in the transfer of specific genes to the bacterial offspring.
It is important to note that extra chromosomal inheritance in bacteria can also involve other cellular structures, such as mitochondria. Mitochondria are organelles found in eukaryotic cells that have their own DNA. In certain cases, the mitochondrial DNA can be inherited independently of the chromosomal DNA, leading to unique inheritance patterns and phenotypes in the offspring.
Studying extra chromosomal inheritance in bacteria is crucial for understanding the mechanisms of genetic transmission, as well as the phenotypic variations that can arise from it. By investigating the transmission of plasmids, bacteriophages, and other genetic elements, researchers can gain insights into the molecular basis of inheritance and the role of mutations in shaping the characteristics of bacterial populations.
Key Concepts | Perspectives |
---|---|
Extra chromosomal inheritance | Antibiotic resistance |
Plasmids | Bacterial conjugation |
Bacteriophages | Mitochondrial inheritance |
Mutations | Phenotypic variations |
Understanding Extra Chromosomal Inheritance in Plants
Extra chromosomal inheritance refers to the transmission of genetic traits through mechanisms that are independent of the chromosomal DNA. In plants, this type of inheritance can occur through the transmission of genes located in organelles such as the mitochondria or chloroplasts. Unlike the chromosomal DNA, which is inherited through sexual reproduction and determines the majority of the organism’s phenotype, extra chromosomal inheritance can result in the inheritance of specific traits that are not determined by the nuclear genome.
Phenotype and Extra Chromosomal Inheritance
Phenotype refers to the observable characteristics of an organism, which are determined by a combination of genetic and environmental factors. While the majority of an organism’s phenotype is determined by the genes found in the chromosomal DNA, extra chromosomal inheritance can play a role in certain traits. For example, traits related to energy production and metabolism, which are influenced by genes in the mitochondria, can be inherited through extra chromosomal mechanisms.
Mutations and Extra Chromosomal Inheritance
Like chromosomal DNA, extra chromosomal DNA can also undergo mutations. These mutations can lead to changes in the traits inherited through extra chromosomal mechanisms. For example, a mutation in a gene located in the mitochondria can affect the energy production of a plant and result in a change in phenotype. These mutations can occur spontaneously or can be induced through various factors such as radiation or chemicals.
The inheritance of these mutated extra chromosomal genes can follow different patterns. Some mutations may not be inherited by the offspring, while others can be inherited in a maternal or paternal manner. The precise mechanisms of the transmission of extra chromosomal mutations in plants are still not fully understood, and further research is needed to elucidate these processes.
In conclusion, extra chromosomal inheritance in plants involves the transmission of genetic traits through mechanisms that are independent of the chromosomal DNA. Genes located in organelles such as the mitochondria can influence specific traits that contribute to the organism’s phenotype. Mutations in these genes can lead to changes in phenotype and can be inherited in different ways. Understanding the intricacies of extra chromosomal inheritance in plants is important for gaining a comprehensive understanding of the genetic mechanisms underlying plant traits and variability.
Implications of Extra Chromosomal Inheritance in Human Health
The study of extra chromosomal inheritance has revealed significant implications for human health. Phenotypic traits that are governed by extra chromosomal inheritance can be passed on to offspring through mechanisms that are distinct from traditional chromosomal inheritance.
One prominent example of extra chromosomal inheritance in humans involves the transmission of traits through the mitochondria. Mitochondria are organelles within cells that have their own DNA and are responsible for producing energy. Mutations in the mitochondrial DNA can lead to the development of various diseases and health conditions.
Unlike chromosomal inheritance, where genetic material is inherited from both parents, extra chromosomal inheritance through mitochondria is solely inherited from the mother. This means that traits or diseases caused by mitochondrial mutations will only be passed on through the maternal line.
Understanding extra chromosomal inheritance is crucial for identifying the causes of certain diseases and conditions. Scientists can study the inheritance patterns of these traits to trace their origins and determine the underlying genetic factors. This knowledge can help in the development of targeted treatments and therapies.
In addition to mitochondria, other extra chromosomal factors, such as cytoplasmic inheritance and epigenetic modifications, also play significant roles in human health. These factors can influence gene expression and contribute to the development of various diseases and conditions.
By studying extra chromosomal inheritance, scientists can gain a deeper understanding of the complex interactions between genes and their environment. This knowledge can provide insights into the mechanisms underlying diseases and contribute to advancements in personalized medicine.
In conclusion, extra chromosomal inheritance has important implications for human health. The transmission of traits and diseases through mitochondria and other extra chromosomal factors can affect the phenotype of individuals and their offspring. Understanding the role of extra chromosomal inheritance in human health is essential for diagnosing, treating, and preventing various diseases and conditions.
The Role of Extra Chromosomal DNA in Disease
Extra chromosomal DNA, also known as extrachromosomal elements (ECEs), plays a significant role in disease development and progression. Unlike the genes present in the chromosomes, ECEs are not inherited from both parents, but rather are acquired during an organism’s lifetime. These genetic elements can have a profound impact on an organism’s phenotype, including their susceptibility to certain diseases.
One example of extra chromosomal inheritance is the transmission of mitochondrial DNA (mtDNA) from the mother to her offspring. Mitochondria are organelles responsible for producing energy within the cell, and they contain their own set of genes separate from those in the chromosomes. Mutations in mtDNA can lead to impaired mitochondrial function, resulting in a range of diseases such as mitochondrial myopathy, encephalopathy, lactic acidosis, and stroke-like episodes (MELAS syndrome).
Unlike chromosomal inheritance, the transmission of extra chromosomal DNA can exhibit unique patterns. For instance, when a mother carries a disease-causing mutation in her mtDNA, all of her children will inherit the mutation. However, the severity of the disease can vary between individuals, as the threshold for symptoms to manifest may differ depending on the amount of mutated mtDNA inherited. This phenomenon is known as heteroplasmy.
In addition to mtDNA, other types of extra chromosomal DNA have been associated with disease. For example, extrachromosomal circular DNA (eccDNA) has been found to contribute to cancer development and drug resistance. EccDNA is an extrachromosomal element that is circular and distinct from the linear DNA strands present in the chromosomes. It can contain oncogenes or drug resistance genes, which can be amplified and integrated into the host genome, contributing to the progression of cancer and resistance to therapy.
Understanding the role of extra chromosomal DNA in disease is crucial for developing effective diagnostic and therapeutic strategies. By identifying specific genetic elements and their impact on disease development, researchers can potentially target these elements with precision therapies aimed at preventing or mitigating disease progression.
In summary:
- Extra chromosomal DNA plays a significant role in disease development and progression.
- Transmission of mitochondrial DNA (mtDNA) can lead to mitochondrial disorders.
- Heteroplasmy can affect disease severity in mtDNA-related diseases.
- Extrachromosomal circular DNA (eccDNA) is associated with cancer development and drug resistance.
- Understanding the role of extra chromosomal DNA is crucial for developing targeted therapies.
Exploring the Potential for Therapeutic Applications
Understanding extra chromosomal inheritance opens up new possibilities for the development of therapeutic applications. This knowledge allows scientists to target specific mutations in the extra chromosomal DNA and develop treatments that can potentially correct these mutations.
Chromosomal mutations can lead to a wide range of genetic disorders and diseases. By studying the extra chromosomal DNA and its transmission patterns, scientists can gain valuable insights into the underlying causes of these disorders and develop targeted therapies to address them.
One area of research that has shown promise is the study of mitochondrial DNA. Mitochondria have their own separate DNA, which is inherited solely from the mother. Mutations in mitochondrial DNA can lead to severe health issues, as they affect the energy production within cells.
By understanding the mechanisms of extra chromosomal inheritance and the transmission of mitochondrial DNA, researchers can develop interventions that target and correct these mutations. This could potentially lead to novel therapeutic approaches for a wide range of diseases and disorders.
Furthermore, the study of extra chromosomal inheritance can also shed light on how certain traits and phenotypes are passed down from one generation to the next. This knowledge can be used in genetic counseling and family planning to provide individuals and families with a better understanding of the potential genetic risks they might face.
In conclusion, the exploration of the potential therapeutic applications of understanding extra chromosomal inheritance holds great promise for the development of targeted treatments for genetic disorders. By studying the transmission patterns of extra chromosomal DNA, researchers can identify and correct mutations, potentially leading to improved health outcomes for individuals and families.
The Impact of Extra Chromosomal Inheritance on Cancer Development
Cancer is a complex disease characterized by uncontrolled cell growth and division. It has long been recognized that genetic factors play a crucial role in cancer development, and the study of chromosomal inheritance has shed light on the transmission of genetic information from one generation to the next. However, recent research has revealed that extra chromosomal inheritance, specifically through mitochondria, can also have significant implications for cancer.
Traditionally, the focus of genetic research has been on the transmission of genes through chromosomes, which are located in the cell nucleus. Mutations in these chromosomal genes can lead to a variety of phenotypic changes, including cancer susceptibility. However, it is now clear that mitochondria, the cell’s powerhouses, also play a role in genetic inheritance.
Mitochondria possess their own small circular DNA molecules, known as mitochondrial DNA (mtDNA), which encode a number of critical genes involved in energy production. Unlike chromosomal DNA, mtDNA is solely inherited from the mother. This maternal transmission of mtDNA can result in the spread of mtDNA mutations through generations. Moreover, cancer cells often exhibit altered mtDNA content and mutations, suggesting that dysfunctional mitochondrial inheritance may contribute to cancer development.
The Role of Extra Chromosomal Inheritance in Cancer
Extra chromosomal inheritance through mitochondria can impact cancer development in several ways. Firstly, mtDNA mutations can lead to impaired mitochondrial function, resulting in compromised energy production and increased oxidative stress. These cellular abnormalities can promote tumor growth and survival, as well as contribute to the development of drug resistance.
Additionally, the phenomenon of heteroplasmy, in which cells harbor a mixture of normal and mutant mtDNA, can contribute to cancer progression. The proportions of mutant mtDNA within cells can vary, and studies have shown that a higher percentage of mutant mtDNA is associated with a more aggressive cancer phenotype.
Implications for Cancer Treatment and Prevention
The recognition of the impact of extra chromosomal inheritance on cancer development opens up new avenues for treatment and prevention. Targeting mitochondrial dysfunction and mtDNA mutations could potentially offer novel therapeutic strategies. Furthermore, understanding the interplay between chromosomal and extra chromosomal inheritance may provide insights into personalized cancer risk assessment and management.
In conclusion, the study of extra chromosomal inheritance, particularly through mitochondria, has revealed important insights into the development and progression of cancer. This knowledge offers new perspectives for understanding the underlying mechanisms of cancer and may lead to innovative approaches in cancer treatment and prevention.

Controversies and Debates in Extra Chromosomal Inheritance
Extra chromosomal inheritance refers to the transmission of genes and phenotypic traits through mechanisms that are separate from the nuclear chromosomes. While the majority of genes are found in the chromosomes within the cell nucleus, there are certain exceptions to this rule.
Mitochondrial Inheritance
One of the most well-known examples of extra chromosomal inheritance is mitochondrial inheritance. Mitochondria, the powerhouses of the cell, contain their own DNA, known as mitochondrial DNA (mtDNA). Unlike nuclear DNA, mtDNA is inherited exclusively from the mother. This means that any mutations in mtDNA can be passed on to offspring from the mother’s side.
However, there is ongoing debate and controversy regarding the extent to which extra chromosomal inheritance through mitochondria affects the phenotype. Some argue that mtDNA mutations have significant effects on phenotypic traits, as mitochondrial function is critical for cellular processes such as energy production. On the other hand, others argue that the contribution of mtDNA mutations to phenotypic variation is minimal, as nuclear genes play a more prominent role in determining phenotype.
Epigenetic Inheritance
Another area of controversy in extra chromosomal inheritance is the role of epigenetic mechanisms. Epigenetic modifications, such as DNA methylation and histone modifications, can alter gene expression without changing the underlying DNA sequence. These modifications can be passed on from one generation to the next, potentially influencing phenotype.
Debates arise over the extent to which epigenetic inheritance contributes to phenotypic variation. Some argue that epigenetic modifications can have profound effects on gene expression and phenotype, with potential implications for evolution and disease. Others argue that the effects of epigenetic modifications are less stable and more variable compared to genetic mutations, and therefore play a less significant role in inheritance.
- In conclusion, the role of extra chromosomal inheritance in shaping phenotype continues to be a topic of debate and controversy. Whether through mitochondrial inheritance or epigenetic mechanisms, these alternative pathways of gene transmission challenge our traditional understanding of chromosomal inheritance. Further research and investigation are needed to fully understand the extent and significance of extra chromosomal inheritance in shaping the traits and characteristics of living organisms.
The Lamarckian Inheritance and Extra Chromosomal DNA
The concept of Lamarckian inheritance proposes that an organism can pass on traits acquired during its lifetime to its offspring. While this theory has been largely discredited in the context of chromosomal inheritance, recent research has shed light on the potential role of extra chromosomal DNA in this process.
Understanding Extra Chromosomal DNA
Extra chromosomal DNA refers to genetic material that is not present within the nuclear chromosomes, but rather exists in organelles such as mitochondria. Mitochondria are responsible for energy production within cells and possess their own set of genes.
Unlike nuclear DNA, which is inherited from both parents, extra chromosomal DNA is typically inherited exclusively from the mother. This uniparental transmission makes it an intriguing area of study when considering the potential inheritance of acquired traits.
Exploring the Role of Extra Chromosomal DNA in Phenotype
Research has indicated that mutations in extra chromosomal DNA can lead to significant changes in phenotype. This suggests that acquired traits associated with mitochondria, and potentially other organelles, could be passed on to offspring.
Recent studies have focused on the transmission of acquired traits through the extra chromosomal DNA in gametes. It is believed that mutations or alterations in the mitochondrial DNA can influence the expression of genes in the nucleus, leading to transgenerational effects on phenotype.
While the exact mechanisms of this process are still being investigated, the potential implications are profound. Understanding the role of extra chromosomal DNA in inheritance could challenge the traditional notion of genetic inheritance and contribute to new perspectives on the transmission of traits from one generation to the next.
In conclusion, the study of the Lamarckian inheritance and extra chromosomal DNA provides a fascinating avenue for exploring non-Mendelian modes of transmission. By unraveling the mechanisms behind the inheritance of acquired traits, scientists can gain a deeper understanding of how genetic information is passed on to offspring and how phenotypic changes can occur across generations.
Contrasting Views on the Role of Extra Chromosomal Inheritance
Extra chromosomal inheritance refers to the transmission of genetic information that is not encoded in the chromosomal DNA. In addition to the chromosomal genes, there is a growing body of evidence suggesting that other cellular components, such as mitochondria, can also contribute to the phenotype of an organism. This alternative form of inheritance challenges the traditional view that only genes located on the chromosomes are responsible for determining the traits of offspring.
The Mitochondrial Perspective
One perspective on extra chromosomal inheritance comes from the study of mitochondria. Mitochondria are unique organelles found in eukaryotic cells that possess their own circular DNA, known as mitochondrial DNA (mtDNA). It has been observed that mutations in mtDNA can lead to a variety of inherited diseases and traits. This suggests that mitochondria can play a significant role in the phenotype of an organism, independent of the genes encoded in the nuclear DNA.
Supporters of the mitochondrial perspective argue that the presence of mtDNA mutations in offspring can be directly linked to the phenotype they exhibit. They believe that the mitochondria can influence various aspects of cellular function, metabolism, and energy production, which in turn can affect the overall development and traits of the organism.
The Gene-Centric View
On the other hand, there are those who adhere to a more gene-centric view of inheritance, focusing primarily on the chromosomal genes. According to this perspective, extra chromosomal inheritance is seen as a secondary mode of inheritance, with the nuclear genes being the primary drivers of trait transmission.
Advocates of the gene-centric view argue that while mitochondrial genes may contribute to the phenotype of an organism, they have a smaller impact compared to the genes on the chromosomes. They contend that the majority of traits are determined by the nuclear DNA, with only a subset of traits being influenced by extra chromosomal factors.
Furthermore, critics of the mitochondrial perspective highlight the numerous complexities associated with studying extra chromosomal inheritance, such as variations in mitochondrial copy number, heteroplasmy, and the potential for nuclear-mitochondrial interactions. They argue that these challenges make it difficult to draw definitive conclusions about the extent and significance of extra chromosomal inheritance in shaping the traits of offspring.
In conclusion, the role of extra chromosomal inheritance in determining the phenotype of offspring is a topic of ongoing debate in the field of genetics. While supporters of the mitochondrial perspective emphasize the importance of factors such as mtDNA mutations, advocates of the gene-centric view place greater emphasis on the chromosomal genes. Both perspectives have their merits and limitations, and further research is needed to fully understand the complex nature of extra chromosomal inheritance.
Exploring the Evolutionary Significance of Extra Chromosomal Inheritance
Extra chromosomal inheritance refers to the transmission of genetic information via organelles outside of the nucleus, such as mitochondria. This type of inheritance has significant implications for the evolution of species, as it can lead to the generation of novel phenotypes and the accumulation of beneficial genetic mutations in offspring.
Role of Mitochondria in Extra Chromosomal Inheritance
Mitochondria, the powerhouses of the cell, play a key role in extra chromosomal inheritance. They have their own circular DNA, separate from the nuclear genome, and replicate independently. During sexual reproduction, mitochondria are typically inherited maternally, meaning they are passed on from the mother to her offspring.
This unique mode of inheritance has important evolutionary consequences. As mitochondria accumulate mutations over time, they can give rise to different mitochondrial lineages within a population. This genetic diversity is advantageous, as it allows populations to adapt to changing environments and increases their chances of survival.
Phenotypic Effects of Extra Chromosomal Inheritance
Extra chromosomal inheritance can also have direct effects on an organism’s phenotype. Mutations in mitochondrial DNA can lead to various metabolic disorders, such as mitochondrial myopathy, Kearns-Sayre syndrome, and Leber’s hereditary optic neuropathy. These disorders are typically characterized by impaired energy production and can have serious consequences for an individual’s health and well-being.
On the other hand, extra chromosomal inheritance can also result in positive phenotypic changes. For example, the presence of certain mitochondrial haplotypes has been associated with increased longevity, improved reproductive success, and resistance to diseases. These advantageous traits can spread through a population via extra chromosomal inheritance, contributing to the evolution and adaptation of species.
Overall, extra chromosomal inheritance, particularly through mitochondria, plays a crucial role in the evolutionary process. It allows for the generation of genetic diversity within populations and can lead to the emergence of both detrimental and beneficial phenotypes. Understanding the mechanisms and implications of extra chromosomal inheritance is therefore essential for comprehending the complexities of genetic inheritance and evolution.
What is extra chromosomal inheritance?
Extra chromosomal inheritance refers to the transmission of genetic information through organelles located outside of the cell nucleus, such as mitochondria or plastids. These organelles contain their own DNA and can pass it on to the next generation.
How is mitochondrial DNA inherited?
Mitochondrial DNA is typically inherited maternally, meaning it is passed on from the mother to her offspring. This is because the egg cell contains mitochondria, while the sperm does not contribute mitochondria to the embryo. Therefore, any genetic disorders or variations in mitochondrial DNA are inherited solely from the mother.
What are some examples of diseases caused by extra chromosomal inheritance?
Some examples of diseases caused by extra chromosomal inheritance include Leber’s hereditary optic neuropathy (LHON), mitochondrial encephalopathy, lactic acidosis, and stroke-like episodes (MELAS), and chronic progressive external ophthalmoplegia (CPEO). These diseases typically affect the organs or tissues that are most dependent on energy produced by mitochondria, such as the eyes, brain, and muscles.
Can extra chromosomal inheritance explain variations in traits among individuals?
Yes, extra chromosomal inheritance can contribute to variations in traits among individuals. This is because mitochondrial DNA can accumulate mutations over time, leading to differences in mitochondrial function and energy production. These variations in energy production can affect various traits and may contribute to differences in metabolism, physical appearance, or susceptibility to certain diseases.
Are there any potential therapeutic approaches for diseases caused by extra chromosomal inheritance?
There are ongoing research efforts to develop potential therapeutic approaches for diseases caused by extra chromosomal inheritance. One approach is the use of gene therapy to introduce healthy copies of mitochondrial genes into affected cells. Another approach is the development of drugs or interventions that can improve mitochondrial function or compensate for the energy deficits caused by mitochondrial mutations. However, more research is needed to fully understand the underlying mechanisms and to develop effective treatments.
Related posts:
- Mitochondrial Genes – Unlocking the Secrets of Cellular Energy and Genetic Inheritance
- Mitochondrial Eve Gene – Unraveling the Ancestry of Modern Humans through Maternal Lineage
- Mitochondrial DNA Testing – Discovering Ancestral Roots Through Genetic Analysis
- Genetic Eve and Adam – Unearthing the Ancestral Origins of Humanity
- Genes That Are Exclusively Inherited from the Mother – Unveiling the Maternally Inherited Genetic Code
- Polg Gene – An In-Depth Analysis of its Function, Significance, and Impact on Human Health
- Unlocking the Mystery – Where Can Genetic Material be Found in the Human Body?
- Location and Distribution of Genes in the Cell – A Comprehensive Guide
- The Impact and Implications of Extra Y Chromosome on Human Development
- Discover Which Genes are Exclusively Inherited from the Mother and the Secrets They Hold
Extrachromosomal inheritance
Cite this chapter.
- Karl Esser 3 &
- Rudolf Kuenen 4
187 Accesses
As early as 1909 Carl Correns , one of the rediscoverers of the Mendelian laws, recognized that the nucleus did not have a monopoly on heredity. He was able to show that the cytoplasm also carries hereditary determinants . In the years following, many cases of extrachromosomal inheritance were described in plants and animals. It became clear that hereditary determinants occurring outside of the chromosomes have the capacity of self-replication and can be transmitted sexually or asexually. Nevertheless, in some cases the phenotypic manifestation of extrachromosomal hereditary determinants depends upon the genotype. The extrachromosomal determinants may reside in the plastids or other cytoplasmic components. While the term genome includes all of the chromosomal hereditary determinants, the extrachromosomal hereditary factors are subdivided into the plastome (factors in the plastids) and the Plasmone (factors in the remainder of the cytoplasm). Since the fungi lack plastids our concern here is with the plasmone.
This is a preview of subscription content, log in via an institution to check access.
Access this chapter
Subscribe and save.
- Get 10 units per month
- Download Article/Chapter or eBook
- 1 Unit = 1 Article or 1 Chapter
- Cancel anytime
- Available as PDF
- Read on any device
- Instant download
- Own it forever
- Compact, lightweight edition
- Dispatched in 3 to 5 business days
- Free shipping worldwide - see info
Tax calculation will be finalised at checkout
Purchases are for personal use only
Institutional subscriptions
Unable to display preview. Download preview PDF.
Arlett , C. F. : Induction of cytoplasmic mutations in Aspergillus nidulans . Nature (Lond.) 179 , 1250–1251 (1957).
Article CAS Google Scholar
— A system of cytoplasmic variation in Aspergillus nidulans . Heredity 15 , 377–388 (1960).
Article Google Scholar
— M. Grindle , and J. L. Jinks : The “red” cytoplasmic variant of Aspergillus nidulans . Heredity 17 , 197–209 (1962).
Article PubMed Google Scholar
Aschan , K. : Studies on dediploidisation mycelia of the basidiomycete Collybia velutipes . Svensk bot. T. 46 , 366–392 (1952).
Google Scholar
Aschan-Åberg , K. : Studies on dedikaryotization mycelia and of F 1 variants in Collybia velutipes . Svensk bot. T. 54 , 311–328 (1960).
Bautz , E. : Beeinflussung der Indophenolblaubildung (Nadi-Reaktion) in Hefezellen durch Röntgenstrahlen. Naturwissenschaften 41 , 375–376 (1954a).
— Untersuchungen über die Mitochondrien von Hefen. Ber. dtsch. bot. Ges. 67 , 281–288 (1954b).
— Mitochondrienfärbung mit Janusgrün bei Hefen. Naturwissenschaften 42 , 49–50 (1955).
—, u. H. Marquardt : Die Grana mit Mitochondrienfunktion in Hefezellen. Naturwissenschaften 40 , 531 (1953a).
— — Das Verhalten oxydierender Fermente in den Grana mit Mitochondrienfunktion der Hefezellen. Naturwissenschaften 40 , 531–532 (1953h).
— — Sprunghafte Änderungen des Verhaltens der Mitochondrien von Hefezellen gegenüber dem Nadi-Reagens. Naturwissenschaften 41 , 121–122 (1954).
Beale , G. H. : The genetics of Paramaecium aurelia. Cambridge (Engl.) 1954.
Beisson-Schecroun , J. : Incompatibilité cellulaire et interactions nucleoplasmiques dans les phénomènes de «barrage » chez le Podospora anserina. Ann. Génét. 4 , 4–50 (1962).
PubMed CAS Google Scholar
Bogen , H. J. , u. G. Kraepelin : Induktion atmungsdefekter Mutanten bei Saccharomyces cerevisiae mit asynchroner und synchronisierter Sprossung. Arch. Mikrobiol. 48 , 291–298 (1964).
Article PubMed CAS Google Scholar
Bulder , C. J. E. A. : Induction of petite mutation and inhibition of synthesis of respiratory enzymes in various yeasts. Antonie v. Leeuwenhoek 30 , 1–9 (1964).
Caspari , E. : Cytoplasmic inheritance. Advanc. Genet. 2 , 1–66 (1948).
Catcheside , D. G. : Introduction to a discussion on the cytoplasm in variation and development. Proc. roy. Soc. B 148 , 285–290 (1958).
Cytoplasmic inheritance. Nature (Lond.) 184 , 1012–1015 (1959).
Chevaugeon , J. , et S. Digbeu : Un second facteur cytoplasmique infectant chez Pestalozzia annulata. C. R. Acad. Sci. (Paris) 251 , 3043–3045 (1960).
CAS Google Scholar
—, et C. Lefort : Sur l’apparition régulière d’un «mutant» infectant chez un champignon du genre Pestalozzia. C. R. Acad. Sci. (Paris) 250 , 2247–2249 (1960).
Correns , C. : Zur Kenntnis der Rolle von Kern und Plasma bei der Vererbung. Z. indukt. Abstamm.- u. Vererb.-L. 2 , 331–340 (1909).
— Nichtmendelnde Vererbung. In: E. Bauer u. M. Hartmann (Hrsg.), Handbuch der Vererbungswissenschaft, Bd. II, S. 1–159. Berlin 1937.
Cuzin , F. : Apparition régulière chez Curvularia pallescenz , d’une variation sectorielle contagieuse, non transmissible par les thallospores. C. R. Acad. Sci. (Paris) 252 , 1656–1658 (1961).
Day , P. R. : A cytoplasmatically controlled abnormality of the tetrades of Copvinus lagopus. Heredity 13 , 81–87 (1959).
Delbrück , M. : In: Unités biologiques douées de continuité génétique: Colloques internat. du CNRS, vol. 7, p. 33, Paris 1949.
Dickson , H. : Observations of inheritance in Copvinus macrorhizus (Pers.) Rea. Ann. Bot. 50 , 719–733 (1936).
Dowding , E. S. , and A. Bakerspigel : Poor fruiters and barrage mutants in Gelasinospora. Canad. J. Bot. 34 , 231–240 (1956).
Ephrussi , B. : Quelques problèmes de la génétique des microorganismes. Arch. Klaus-Stift. Vererb.-Forsch. 26 , 403–425 (1951).
— The interplay of heredity and environment in the synthesis of respiratory enzymes in yeast. Harvey Lect. Series 46 , 45–67 (1952).
— Nucleo-cytoplasmic relations in microorganisms. Oxford (Engl.) 1953.
— Die Bestandteile des cytochrombildenden Systems der Hefe. Naturwissenschaften 43 , 505–511 (1956).
—, and H. Hottinguer: Cytoplasmic constituents of heredity. Cold Spr. Harb. Symp. quant. Biol. 16 , 75–85 (1951).
— — et A. M. Chimènes : Actions de Facriflavine sur les levures. I. La mutation «petite colonie». Ann. Inst. Pasteur 76 , 351–364 (1949a).
— — et H. Roman : Sur le comportement des mutants a déficience respiratoire de la levure dans les croisements. Caryologia Vol. Suppl. 1112–1113 (1954).
— — — Supressiveness: A new factor in the genetic determinism of the synthesis of respiratory enzymes in yeast. Proc. nat. Acad. Sci. (Wash.) 41 , 1065–1071 (1955).
— — et J. Tavlitzki : Action de Facriflavine sur les levures. II. Étude génétique du mutant «petite colonie». Ann. Inst. Pasteur 76 , 419–450 (1949b).
—, et P. P. Slonimski : La synthèse adaptive des cytochromes chez la levure de boulangerie. Biochim. biophys. Acta (Amst.) 6 , 256–267 (1950).
— — Yeast mitochondria, subcellular units involved in the synthesis of respiratory enzymes in yeast. Nature (Lond.) 176 , 1207–1209 (1955).
Esser , K. : Die Incompatibilitätsbeziehungen zwischen geographischen Rassen von Podospora anserina (Ces.) Rehm. II. Die Wirkungsweise der Incompatibilitätsgene. Z. Vererbungsl. 90 , 29–52 (1959).
Faulkner , B. M. , and C. F. Arlett : The “minute” cytoplasmic variant of Aspergillus nidulans. Heredity 19 , 63–73 (1964).
Fink , H. : Klassifizierung von Kulturhefen mit Hilfe des Cytochromspektrums. Hoppe-Seylers Z. physiol. Chem. 210 , 197–219 (1932).
—, u. E. Berwald : Über die Umwandlung des Cytochromspektrums in Bierhefen. Biochem. Z. 258 , 141–153 (1933).
Fitzgerald , P. H. : Genetic and epigenetic factors controlling female sterility in Neurospora crassa. Heredity 18 , 47–62 (1963).
Fries , N. , and K. Aschan : The physiological heterogeneity of the dikaryotic mycelium of Polyporus abietinus investigated with the aid of micrurgical technique. Svensk bot. T. 46 , 429–445 (1952).
Gowdridge , B. M. : Heterocaryons between strains of Neurospora crassa with different cytoplasms. Genetics 41 , 780–789 (1956).
Grenson , M. : A gene-induced cytoplasmic mutation in yeast. Proc. XI. intern. Congr. of Genetics, vol. 1, p. 202, The Hague 1963.
Grindle , M. : Nucleo-cytoplasmic interactions in the “red” cytoplasmic variant of Aspergillus nidulans. Heredity 19 , 75–95 (1964).
Hagemann , R. : Plasmatische Vererbung. Jena 1964.
— Extrachromosomale Vererbung. In: Bünning , E. et al. (Hrsg.): Fortschritte der Botanik, Springer: Berlin-Heidelberg-New York 1966, pp. 202–216.
Harder , R. : Zur Frage nach der Rolle von Kern und Protoplasma im Zellgeschehen und bei der Übertragung von Eigenschaften. Z. Bot. 19 , 337–407 (1927 a).
— Über mikrochirurgische Operationen an Hymenomyceten. Z. wiss. Mikr. 44 , 173–182 (1927b).
Hardesty , B. A. , and H. K. Mitchell : The accumulation of free fatty acids in poky, a maternal inherited mutant of Neurospora crassa. Arch. Biochem. 100 , 330–334 (1963).
Hartman , P. E. , and C. J. Liu : Comparative cytology of wild type Saccharomyces and a respirationally deficient mutant. J. Bact. 67 , 77–85 (1954).
Haskins , F. A. , A. Tissières , H. K. Mitchell , and M. B. Mitchell : Cytochromes and the succinic acid oxidase system of poky strains of Neurospora. J. biol. Chem. 200 , 819–826 (1953).
Jacob , F. , P. Schaeffer , and E. L. Wollman : Episomic elements in bacteria. Microbiol. Genetics, X. Symp., pp. 67–91, London 1960.
Jinks , J.L. : Somatic selection in fungi. Nature (Lond.) 174 , 409–410 (1954).
— Naturally occurring cytoplasmic changes in fungi. C. R. Lab. Carlsberg, Sér. Physiol. 26 , 183–203 (1956).
— Selection for cytoplasmic differences. Proc. roy. Soc. B 146 , 527–540 (1957).
— Cytoplasmic differentiation in fungi. Proc. roy. Soc. B 148 , 314–321 (1958).
Johnson , T. : Variation and the inheritance of certain characters in rust fungi. Cold Spr. Harb. Symp. quant. Biol. 11 , 85–93 (1946).
Joly , P. : Données récentes sur la génétique des champignons supérieurs (Ascomycètes et Basidiomycètes). Rev. Mycol. (Paris) 29 , 115–186 (1964).
Kimura , K. : On the diploidization by the double compatible diploid mycelium in the hymenomycetes. Bot. Mag. (Tokyo) 67 , 238–242 (1954).
Kraepelin , G. : Normalisierung des Atmungsdefektes bei Hefe. Rückführung stabilisierter RD -Mutanten in voll atmungsfähige Normalzellen. Arch. Mikrobiol. 48 , 299–305 (1964).
L’Héritier , P. : The CO 2 sensitivity problem in Drosophila. Cold Spr. Harb. Symp. quant. Biol. 16 , 99–112 (1951).
—Le problème de l’hérédité non chromosomique. Ann. Biol. 1 , 3–34 (1962).
Lindberg , G. D. : A transmissible disease of Helminthosporium victoriae. Phytopathology 49 , 29–32 (1959).
Lindegren , C. C. : Cytoplasmic inheritance. Ann. N.Y. Acad. Sci. 68 , 366–379 (1957).
Mahony , M. , and D. Wilkie : An instance of cytoplasmic inheritance in Aspergillus nidulans. Proc. roy. Soc. B 148 , 359–361 (1958).
— — Nucleo-cytoplasmic control of perithecial formation in Aspergillus nidulans. Proc. ryo. Soc. B 156 , 524–532 (1962).
Marcou , D. : Sur la longévité des souches de Podospora anserina cultivées à divers températures. C. R. Acad. Sci. (Paris) 239 , 895–897 (1954a).
— Sur le rajeunissement par le friod des souches de Podospora anserina. C. R. Acad. Sci. (Paris) 239 , 1153–1155 (1954b).
— Rajeunissement et arrêt de croissance chez Podospora anserina. C. R. Acad. Sci. (Paris) 244 , 661–663 (1957).
— Sur la déterminisme de la sénescence observée chez l’ascomycète Podospora anserina. Proc. X. intern. Congr. of Genetics, vol. II, p. 179, Montreal 1958.
— Notion de longévité et nature cytoplasmique de déterminent de la sénescence. Ann. Sci. nat. Bot. 2 , 653–764 (1961).
—, et J. Schecroun : La sénescence chez Podospora anserina pourrait être due à des particules cytoplasmiques infectantes. C. R. Acad. Sci. (Paris) 248 , 280–283 (1959).
Marquardt , H. : Die Natur der Erbträger im Zytoplasma. Ber. dtsch. bot. Ges. 65 , 198–217 (1952).
—, u. E. Bautz : Die Wirkung einiger Atmungsgifte auf das Verhalten von Hefe-Mitochondrien gegenüber der Nadi-Reaktion. Naturwissenschaften 41 , 361–362 (1954).
Mather , K. : Nucleus and cytoplasm in heredity and development. Proc. roy. Soc. B 148 , 362–369 (1958).
Michaelis , P. : Interactions between genes and cytoplasm in Epilobium. Cold Spr. Harb. Symp. quant. Biol. 16 , 121–129 (1951).
— Cytoplasmic inheritance in Epilobium and its theoretical significance. Advanc. Genet. 6 , 287–401 (1954).
— Genetical interactions between nucleus and cytoplasmic cell constituents. Path. et Biol. 9 , 769–772 (1961).
Mitchell , M. B. , and H. K. Mitchell : A case of ‘‘maternal’’ inheritance in Neurospora crassa. Proc. nat. Acad. Sci. (Wash.) 38 , 442–449 (1952).
— —, and A. Tissières : Mendelian and non-Mendelian factors affecting the cytochrome system in Neurospora crassa. Proc. nat. Acad. Sci. (Wash.) 39 , 606–613 (1953).
Muneta , J. , and A.M. Srb : Paternal transmission of extra-chromosomal properties in Neurospora. IX. Intern. Bot. Congr., vol. II, p. 274, Montreal (Canada) 1959.
Nanney , D. L. : The role of cytoplasm in heredity. In: W. D. McElroy and B. Glass (edits.), The chemical basis of heredity, pp. 134–166. Baltimore 1957.
Oehlkers , F. : Neue Überlegungen zum Problem der außerkaryotischen Vererbung. Z. indukt. Abstamm.- u. Vererb.-L. 84 , 213–250 (1952).
Oehlkers , F. — Außerkaryotische Vererbung. Naturwissenschaften 40 , 78–85 (1953).
Papazian , H. P. : Sectoring variants in Schizophyllum . Amer. J. Bot. 42 , 394–400 (1955).
— Sex and cytoplasm in the fungi. Trans. N.Y. Acad. Sci. 18 , 388–397 (1956).
— The genetics of basidiomycetes. Advanc. Genet. 9 , 41–69 (1958).
Quintanilha , A. , et S. Balle : Étude génétique des phénomènes de nanisme chez les hymenomycètes. Boll. Soc. Brot. 14 , 17–46 (1940).
Raut , C. : Cytochrome deficient yeast strains. Genetics 36 , 572 (1951).
— A cytochrome deficient mutant of S aecharomyces cerevisiae . Exp. Cell Res. 4 , 295–305 (1953).
— Heritable non-genic changes induced in yeast by ultraviolet light. J. cell. comp. Physiol. 44 , 463–475 (1954).
Renner , O. : Die pflanzlichen Piastiden als selbständige Elemente der genetischen Konstitution. Ber. sächs. Akad. Wiss. Leipzig, math.-physik. Kl. 86 , 241–266 (1934).
Rhoades , M. M. : Interaction of genie and non-genic hereditary units and the physiology of non-genic inheritance. In: W. Ruhland (Hrsg.), Handbuch der Pflanzenphysiologie, Bd. I, S. 19–57. Berlin-Göttingen-Heidelberg 1955.
Rizet , G. : Les phénomènes de barrage chez Podospora anserina . I. Analyse génétique des barrages entre souches S et s. Rev. Cytol. Biol, végét. 13 , 51–92 (1952).
— Sur la multiplicité des mécanismes génétiques conduisant à des barrages chez Podospora anserina . C. R. Acad. Sci. (Paris) 237 , 666–668 (1953a).
— Sur l’impossibilité d’obtenir la multiplication végétative interrompue et illimitée de l’ascomycète Podospora anserina . C. R. Acad. Sci. (Paris) 237 , 838–840 (1953b).
— Sur la longévité des souches de Podospora anserina . C. R. Acad. Sci. (Paris) 237 , 1106–1109 (1953 c).
— Les modifications qui conduisent à la sénescence chez Podospora sontelles de nature cytoplasmique ? C. R. Acad. Sci. (Paris) 244 , 663–665 (1957).
—, et D. Marcou : Longévité et sénescence chez l’acsomycète Podospora anserina . Compt. rend. VIII. Congr. intern. Bot. Sect., vol. 10, pp. 121–128, Paris 1954.
— — et J. Schecroun : Deux phénomènes d’héridité cytoplasmique chez l’ascomycète Podospora anserina . Bull. Soc. Franc. physiol. végét. 4 , 136–149 (1958).
—, et J. Schecroun : Sur les facteurs cytoplasmiques associés ou couple des gènes S— s chez Podospora anserina . C. R. Acad. Sci. (Paris) 249 , 2392–2394 (1959).
Roper , J. A. : Nucleo-cytoplasmic interactions in Aspergillus nidulans . Cold Spr. Harb. Symp. quant. Biol. 23 , 141–154 (1958).
Sager , R. , and F. J. Ryan : Cell heredity. New York and London 1961.
Schatz , G. , H. Tuppy u. J. Klima : Trennung und Charakterisierung cytoplasmatischer Partikel aus normaler und atmungsdefekter Bäckerhefe. Ein Beitrag zur Frage der genetischen Kontinuität der Mitochondrien von S aecharomyces cerevisiae . Z. Naturforsch. 18b , 145–153 (1963).
Schecroun , J. : Sur la réversion provoquée des souches s S en souches s chez Podospora anserina . C. R. Acad. Sci. (Paris) 246 , 1268–1270 (1958a).
— Sur la réversion provoquée d’une modification cytoplasmique chez Podospora anserina . Proc. X. intern. Congr. Genetics, vol. II, pp. 252–253, Montrale (Canada) 1958b.
— Sur la nature de la différence cytoplasmique entre souches s et s S de Podospora anserina . C. R. Acad. Sci. (Paris) 248 , 1394–1397 (1959).
Sharpe , S. : A closed system of cytoplasmic variation in Aspergillus glaucus . Proc. roy. Soc. B 148 , 355–359 (1958).
Sherman , F. : Respiration-deficient mutants of yeast. I. Genetics. Genetics 48 , 375–385 (1963).
— Mutants of yeast deficient in cytochrome C. Genetics 49 , 39–48 (1964).
Silagi , S. : Interactions between a cytoplasmic factor and nuclear genes in Neurospora crassa . Proc. XL intern. Congr. Genetics, vol. 1, pp. 202, The Hague 1963.
Slonimski , P. P. : Action de Facriflavine sur les levures. IV. Mode d’utilisation de glucose par les mutants «petite colonie». Ann. Inst. Pasteur 76 , 510–530 (1949a).
— Action de Facriflavine sur les levures. VIL Sur l’activité catalytique du cytochrome C des mutants «petite colonie» de la levure. Ann. Inst. Pasteur 77 , 774– 777 (1949b).
— Effet de l’oxygène sur la formation de quelques enzymes chez le mutant «petite colonie» de S aechar omyces cerevisiae . C. R. Acad. Sci. (Paris) 231 , 375–376 (1950).
— Recherches sur la formation des enzymes réspiratoires chez la levure. Thèse, Fac. des Sci. Paris 1952.
—, et B. Ephrussi : Action de l’acriflavines sur les levures. V. Le système de cytochromes des mutants «petite colonie». Ann. Inst. Pasteur 77 , 47–63 (1949).
— et H. M. Hirsch : Nouvelles données sur la constitution enzymatique du mutant «petite colonie» de S aechar omyces cerevisiae . C. R. Acad. Sci. (Paris) 235 , 741–743 (1952).
Sonneborn , T. M. : Kappa and related particles in Paramecium . Advanc. Virus Res. 6 , 231–356 (1959).
Srb , A. M. : Some consequences of nuclear cytoplasmic recombinations among various Neurosporas . Cold Spr. Harb. Symp. quant. Biol. 23 , 269–277 (1958).
Stubbe , W. : Genetische Analyse des Zusammenwirkens von Genom und Plasmon bei Oenothera . Z. Vererbungsl. 90 , 288–298 (1959).
— Extrem disharmonische Genom-Plastom-Kombinationen und väterliche Piastidenvererbung bei Oenothera . Z. Vererbungsl. 94 , 392–411 (1963).
Tavlitzki , J. : Action de Facriflavine sur les levures. III. Étude de la croissance des mutants «petite colonie». Ann. Inst. Pasteur 76 , 497–509 (1949).
Vandendries , R. : La tétrapolarité sexuelle de Pleurotus colombinus . Cellule 41 , 267–278 (1932).
Wettstein , F. v.: Die genetische und entwicklungsphysiologische Bedeutung des Cytoplasmas. Z. indukt. Abstamm.- u. Vererb.-L. 73 , 349–366 (1937).
Windisch , S. : Über Bildung und Bedeutung plasmatischer Mutanten von Kulturhefen. Brauerei, Wiss. Beil. 11 , 3–7 (1958).
Ycas , M. , and T. J. Starr : The effect of glycine and protoporphyrin on a cytochrome deficient yeast. J. Bact. 65 , 83–88 (1953).
Yotsuyanagi , Y. : Mitochondria and refractive granules in the yeast cells. Nature (Lond.) 176 , 1209 (1955).
— Études sur le chondriome de la levure. II. Chondriomes des mutants à déficience respiratoire. J. Ultrastruct. Res. 7 , 141–158 (1962).
References which have come to the authors’ attention after conclusion of the German manuscript A
Beale , G. H. : The role of the cytoplasm in heredity. Proc. roy. Soc. B 164 , 209–218 (1966).
Sager , R. : Mendelian and non-Mendelian heredity: a reappraisal. Proc. roy. Soc. B 164 , 290–297 (1966).
—, and Z. Ramanis : Recombination of nonchromosomal genes in Chlamydomonas . Proc. nat. Acad. Sci. (Wash.) 53 , 1053–1061 (1965).
Srb , A. M. : Extrachromosomal heredity in fungi. Reproduction: Molecular, subcellular and cellular, p. 191–211. New York 1965.
Avers , C. J. , and C. D. Dryfuss : Influence of added nucleosides on acriflavin induction of petite mutants in baker’s yeast. Nature (Lond.) 206 , 850 (1965).
— C. R. Pfeffer , and M. W. Rancourt : Acriflavine induction of different kinds of “petite” mitochondrial populations in Saccharornyces cerevisiae . J. Bact. 90 , 481–494 (1965).
Ephrussi , B. , et S. Grandchamp : Études sur la suppressivité des mutants à déficience respiratoire de la levure. I. Existence au niveau cellulaire de divers “degrés de suppressivité”. Heredity 20 , 1–7 (1965).
— H. Jakob et S. Grandchamp : Études sur la suppressivité des mutants à déficience respiratoire de la levure. II. Étapes de la mutation grande en petite provoquée par le facteur suppressif. Genetics 54 , 1–29 (1966).
Horn , P. , and D. Wilkie : Selective advantage of the cytoplasmic respiratory mutant of S aecharomyces cerevisiae in a cobalt medium. Heredity 21 , 625–635 (1967).
Jakob , H. : Complementation entre mutants à déficience respiratoire de Saccharomyces cerevisiae : Établissement et régulation de la respiration dans les zygotes et dans leur proche descendence. Genetics 52 , 75–98 (1965).
Kraepelin , G. : Zur Wirkung von 2,4-Dinitrophenol und Acridinorange auf Saccharomyces cerevisiae und dessen atmungsdefekte Mutante Mk. Arch. Mikrobiol. 50 , 52–58 (1965).
— Der Einfluß der Vorkultur auf die Induktion atmungsdefekter Hefemutanten durch Acridinorange. Arch. Mikrobiol. 50 , 59–62 (1965).
— Synchrone Vorgänge in asynchron sprossenden Saccharomyces- Kulturen: Mutationsauslösung und Abtötung durch Acridine. Arch. Mikrobiol. 50 , 63–67 (1965).
Morita , T. , and I. Mifuchi : Effect of methylene blue on the action of 4-nitroquinoline N-oxide and acriflavine in inducing respiration-deficient mutants of Saccharomyces cerevisiae . Jap. J. Microbiol. 9 , 123–129 (1965).
Moustacchi , E. , et H. Marcovich : Induction de la mutation «petite colonie» chez la levure par le 5-flurouracile. C. R. Acad. Sci. (Paris) 256 , 5646–5648 (1963).
—, and D. H. Williamson : Physiological variations in satellite components of yeast DNA detected by density gradient centrifugation. Biochem. biophys. Res. Commun. 23 , 56–61 (1966).
Sugimura , T. , K. Okabe , M. Nagao , and N. Gunge : A respiration-deficient mutant of Saccharomyces cerevisiae which accumulates porphyrins and lacks cytochromes. Biochim. biophys. Acta (Amst.) 115 , 267–275 (1966).
Tuppy , H. , E. Haslbrunner u. G. Schatz : Extranukleare Desoxyribonukleinsäure in aerob und anaerob gezüchteter normaler Bäckerhefe sowie in der atmungsdefizienten „petite“ -Mutante. Mh. Chem. 96 , 1831–1841 (1965).
Wintersberger , E. : Synthesis and function of mitochondrial ribonucleic acid. In: J. M. Tager, S. Papa, E. Quagliariello, and E. C. Slater: (eds.): Regulation of metabolic processes in mitochondria. Amsterdam 1966.
—, u. H. Tuppy : DNA-abhängige RNA-Synthese in isolierten Hefe-Mitochondrien. Biochem. Z. 341 , 399–408 (1965).
Heller , J. , and E. L. Smith : The amino acid sequence of cytochrome C of Neurospora crassa . Proc. nat. Acad. Sci. (Wash.) 54 , 1621–1625 (1965).
McDougall , K. J. , and T. H. Pittenger : A cytoplasmic variant of Neurospora crassa . Genetics 54 , 551–565 (1966).
Munkres , K. D. , and D. O. Woodward : On the genetics of enzyme locational specificity. Proc. nat. Acad. Sci. (Wash.) 55 , 1217–1224 (1966).
Silagi , S. : Interactions between an extrachromosomal factor, poky , and nuclear genes in Neurospora crassa . Genetics 52 , 341–347 (1965).
Srb , A. M. : Extrachromosomal factors in the genetic differentiation of Neurospora . Symp. Soc. Experim. Biol. XVII, Cell Different. 1963, p. 175–187.
Arlett , C. F. : The radiation sensitivity of a cytoplasmic mutant of Aspergillus nidulans . Int. J. Radiat. Biol. 10 , 539–549 (1966).
Chevaugeon , J. : Modification extra-chromosomique et âge du thalle chez le Pestalozzia annulata . C. R. Acad. Sci. (Paris) 255 , 1980–1982 (1962).
— Conditions de la différenciation du mycélium modifié chez le Pestalozzia annulata . C. R. Acad. Sci. (Paris) 255 , 3450–3452 (1962).
Chevaugeon , J. : Une période d’incubation s’interpose entre deux événe ments impliqués dans la modification extra-chromosomique du Pestalozzia annulata . C.R. Acad. Sci. (Paris) 257 , 217–220 (1963).
— Modification expérimentale du phénotype normal chez le Pestalozzia annulata . C. R. Acad. Sci. (Paris) 261 , 2730–2733 (1965).
— Mise en évidence de mécanismes de répression de la variation extrachromosomique chez le Pestalozzia annulata . C. R. Acad. Sci. (Paris) 263 , 120–123 (1966).
—, et L. Clouet : Temps et lieu des deux événements aléatoires impliqués dans la modification extra-chromosomique du Pestalozzia annulata . C. R. Acad. Sci. (Paris) 256 , 4068–4071 (1963).
— L. Clouet et G. Michel : Nutrition, croissance et modification extrachromosomique du Pestalozzia annulata . C. R. Acad. Sci. (Paris) 261 , 517–520 (1965).
Croft , J. H. : A reciprocal phenotypic instability affecting development in Aspergillus nidulans . Heredity 21 , 565–579 (1967).
Gregory , K. F. , and J. C. C. Huang : Tyrosinase inheritance in Streptomyces scabies . I. Genetic recombination. J. Bact. 87 , 1281–1286 (1964).
— — Tyrosinase inheritance in Streptomyces scabies . II. Induction of tyrosinase deficiency by acridine dyes. J. Bact. 87 , 1287–1294 (1964).
Kerr , S. : Disappearance of a genetic marker from a cytoplasmic hybrid Plasmodium of a true slime mold. Science 147 , 1586–1588 (1965).
Download references
Author information
Authors and affiliations.
Ruhr-Universität Bochum, Germany
Dr. phil. Karl Esser ( o. Professor )
Köln, Germany
Dr. rer. nat. Rudolf Kuenen ( Oberstudienrat )
You can also search for this author in PubMed Google Scholar
Rights and permissions
Reprints and permissions
Copyright information
© 1967 Springer-Verlag Berlin · Heidelberg
About this chapter
Esser, K., Kuenen, R. (1967). Extrachromosomal inheritance. In: Genetics of Fungi. Springer, Berlin, Heidelberg. https://doi.org/10.1007/978-3-642-86814-6_8
Download citation
DOI : https://doi.org/10.1007/978-3-642-86814-6_8
Publisher Name : Springer, Berlin, Heidelberg
Print ISBN : 978-3-642-86816-0
Online ISBN : 978-3-642-86814-6
eBook Packages : Springer Book Archive
Share this chapter
Anyone you share the following link with will be able to read this content:
Sorry, a shareable link is not currently available for this article.
Provided by the Springer Nature SharedIt content-sharing initiative
- Publish with us
Policies and ethics
- Find a journal
- Track your research
- BiologyDiscussion.com
- Follow Us On:
- Google Plus
- Publish Now
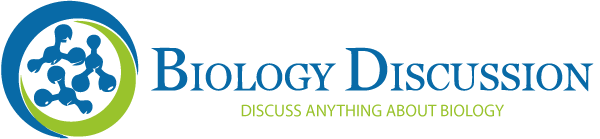
Term Paper on Inheritance | Genetic Attribute | Biology
ADVERTISEMENTS:
Here is a compilation of term papers on ‘Inheritance’ for class 9, 10, 11 and 12. Find paragraphs, long and short term papers on ‘Inheritance’ especially written for school and college students.
Term Paper on Inheritance
Term Paper Contents:
- Term Paper on Genetic Engineering
ADVERTISEMENTS: (adsbygoogle = window.adsbygoogle || []).push({}); 1. Term Paper on Variation in Offspring :
Sexual reproduction leads to variation in the offspring, that is, each individual has different characteristics. No two offspring from the same parents, produced by sexual reproduction, are genetically identical. An exception occurs when the offspring develop from the same ovum and sperm, in which case they are ‘identical twins’.
Two types of variation are seen:
A. Continuous, and
B. Discontinuous.
A. Continuous Variation :
Continuous variation is the result of the interaction of two factors:
(i) The genes that are inherited by an individual
(ii) The effect of the environment on the individual.
The environmental factors involved might include:
(i) The availability and type of food (in animals)
(ii) Disease
(iii) The Climate:
a. Amount of sunlight
b. Temperature
c. Amount of available water.
(iv) The ions present in the soil (in plants)
(v) Competition from other organisms in the environment.
In continuous variation, individuals show a range between the two extremes. Every possible form between the two extremes will exist.
Examples of continuous variation are:
(i) Body mass
(ii) Height
(iii) Foot size.
B. Discontinuous Variation :
This is the result of inheritance only. There are few types, with no intermediates.
Examples of discontinuous variation are:
(i) Blood groups
(ii) The ability to roll the tongue into a ‘U’ shape (either you can or you cannot!).

2. Term Paper on the Chemical Structure of Chromosomes :
Chromosomes, situated in the nuclei of all living cells (except bacteria, which have no true nucleus) are made of the chemical substance DNA. The DNA molecule, looking rather like a very long, twisted rope ladder, is made up of two strands (of alternating sugar and phosphate units) held together by pairs of chemical units called bases (the rungs of the ladder – see the upper section of the diagram below). The molecule is described as a double helix (Greek ‘helix’ = a spiral).
There are four bases only:
A (Adenine)
C (Cytosine)
G (Guanine)
T (Thymine)
These bases link with one another in the following ways (‘The Rule of Base Pairing’)

3. Term Paper on the Unit of Inheritance :
All living organisms manufacture proteins in their cells. These are used either for structural purposes within the cell or as enzymes to control chemical processes in the cell. All proteins are made up of linked amino acids.
The sequence of bases (e.g. CATGCTAGCCTA) on one of the two strands is a code. When protein molecules are made in the cytoplasm of a cell, a copy of the bases on a section of a DNA strand is made and passed out into the cytoplasm of the cell. The sequence of bases is first split into triplets (CAT, GCT, AGC, and CTA). Each triplet is then responsible for lining up one particular amino acid in the sequence of amino acids that will link to form a protein. Each of the 22 amino acids has its own triplet.
Since the sequence of bases on DNA molecules is different for each (sexually produced) individual, it follows that no two individuals will make protein molecules with exactly the same sequence of amino acids. The length of chromosome which contains the bases necessary to make one protein molecule is otherwise known as a gene.
Definition of Gene:
A Gene is defined as a unit of inheritance.
For the purpose of understanding the mechanism of simple inheritance, it is convenient to imagine a chromosome as a string of beads, like that shown below, each bead represents one gene.

During cell division, genes are copied and these copies are passed on from parent to offspring via chromosomes in the nuclei of the parents’ gametes.
4. Term Paper on Genetic Inheritance :
Every member of the same species has the same number of chromosomes in each (healthy) cell of their body. These chromosomes exist in matching pairs. For example, human beings have 23 matching or homologous pairs of chromosomes, a total number of 46. Of each pair of matching chromosomes, one is inherited from a person’s mother and one is inherited from their father.
The genes of homologous chromosomes also match. In other words, if we look at two strings of beads, like those shown in Fig. 79, the order of the different shapes of beads is the same on both strings.
Matching genes on homologous chromosomes are called alleles.

You can see in Fig. 79 that a pair of beads (like the two ■ shown at position 1) always match in shape, but do not always match in colour. This is a way of showing that one pair of allele’s controls one character, but each allele may exist in two forms- they may be dominant or recessive.
In Fig. 79, the alleles in position 1 are both dominant, in position 2 they are both recessive and in position 3, there is one of each.
For a particular character, an offspring may therefore inherit either:
i. Two dominant alleles, one from each parent. The offspring is described as homozygous dominant.
ii. Two recessive alleles, one from each parent. The offspring is described as homozygous recessive.
iii. One dominant and one recessive allele. The offspring is described as heterozygous.
These are the three possible genotypes of the individual.
If at least one dominant allele is present in the genotype, the individual will show the dominant feature in their appearance (or phenotype). Thus the homozygous dominant and heterozygous genotypes will give the same phenotype. The homozygous recessive individual will have the alternative (or ‘contrasting’) phenotype.
5. Term Paper on Variation as a Result of Mutation :
Genes and chromosomes are always subject to change (or mutation) as a result of environmental forces acting upon them. These forces are known as mutagens, and include X-rays, atomic radiation, ultraviolet light and some chemicals. Exposure to higher doses of any of these mutagens will lead to a greater rate of mutation.
Definition of Mutation:
A mutation is a spontaneous change in the structure of a gene or chromosome.
Gene Mutation:
Sickle-cell anaemia is an example of a condition caused by a gene mutation.
Both parents pass on a mutated (and recessive) allele for making haemoglobin in red blood cells. The homozygous recessive offspring cannot make effective haemoglobin, and cannot carry sufficient oxygen in their blood. Their red blood cells also take on a distorted shape. A person with this condition is likely to die at an early age.
Chromosome Mutation :
Down’s syndrome is an example of a condition caused by a chromosome mutation.
As described above, there are 46 (23 pairs of) chromosomes in every normal cell of the human body; there are 23 unpaired chromosomes in each gamete. Forty-six is known as the diploid number and 23 as the haploid number.
If, in the production of gametes by one of the parents, one extra chromosome enters one of the gametes, then there will be 24 (instead of 23) chromosomes in that gamete. If this gamete is involved in the process of fertilisation, there will be 47 (instead of 46) chromosomes in the zygote. In older parents, there is a greater tendency for chromosome number 21 not to separate properly as gametes are being made.
A child who inherits the extra chromosome will suffer from Down’s syndrome. Their physical and mental development will be slow, and they will have a distinctive facial appearance.
6. Term Paper on Monohybrid Inheritance :
Organisms inherit alleles for thousands of different contrasting characters, for example, human hair is either curly or straight, and we either can or cannot smell the scent of certain flowers. Monohybrid inheritance refers to only one pair of contrasting characters, such as curly or straight hair, controlled in the individual by one pair of alleles.
There are two types of monohybrid inheritance:
A. With complete dominance, and
B. With co-dominance.
A. With Complete Dominance :
This is where the presence of only one dominant allele will decide the appearance (or phenotype) of the individual.
Example: Coat colour in mice.
In mice, brown coat colour is dominant over grey coat colour. In an experiment, a homozygous dominant (or ‘pure-breeding’) brown male mouse mated with a homozygous recessive (also pure-breeding) grey female mouse. All their offspring (that is, the F 1 or first filial generation) were found to be brown.
The offspring of the F 1 generation were then allowed to freely interbreed. It was found that their offspring (the F 2 generation) were brown to grey in a 3: 1 ratio.
This can be explained in a genetic diagram, set out below:
Genetic Diagrams :
Genetic diagrams are a way of looking at the combinations of alleles produced by two parents. In constructing genetic diagrams, the letters of the alphabet (rather than beads) are used to represent alleles. A dominant allele is represented by a capital letter (like A, B, C) and its recessive allele is represented by a small (or lower case) version of the same letter (like a, b, c).
For Example:

(Note: Statistically, there is an exactly equal chance of either of the alleles from the male combining with either of the alleles from female at the time of fertilisation.)

The results are given as a statistical ratio in a large sample. The smaller the sample, the less likely that the ratios will be the same as shown.
In humans, where only one offspring is likely to be produced at a time, the probability of that offspring inheriting a particular feature is often given. Probability is usually expressed as a percentage.
Example: Cystic fibrosis in humans
Cystic fibrosis is an inherited condition that affects the type of mucus found in people’s lungs. Most people produce normal protein in the mucus of their lungs. They possess at least one dominant allele, which may be called ‘F’. The homozygous recessive person, suffering from cystic fibrosis, has the genotype ‘ff’. Their lungs contain particularly thick and sticky mucus, which makes gaseous exchange difficult.
Genetic Diagram: Both Parents Heterozygous for Cystic Fibrosis:
In the diagram below, there are two parents who are both heterozygous for cystic fibrosis (their genotype is ‘Ff’). If they have a child, the probability of this child having the genotype ff, and therefore suffering from cystic fibrosis, is 25%.
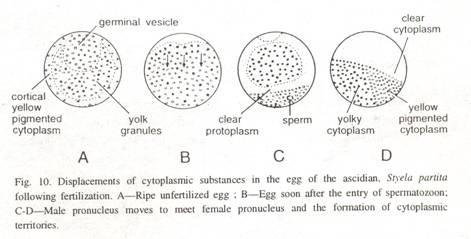
This page has been archived and is no longer updated
Developing the Chromosome Theory

Early Breakthroughs: Mendel and Darwin Propose Mechanisms of Heredity
Flemming, boveri, and sutton connect chromosomes to heredity, walther flemming describes chromosomes.
By the middle of the nineteenth century, scientists understood that cells derived from other cells and that the hereditary information was located in the nucleus, but the physical nature of the hereditary material remained unknown. The microscopes of the time provided very poor resolution of living cellular structures, making it necessary for investigators to treat fixed cells with various stains to enhance the contrast of their contents. Thus, using innovative microscopy techniques and painstaking precision, German anatomist Walther Flemming recognized and explored the fibrous network within the nucleus, which he termed chromatin , or "stainable material." (Flemming had actually discovered the chromosome , although the term would be coined a few years later by Heinrich Waldeyer.) Flemming noted that during cell division, the chromatin formed threadlike bodies, which he termed mitosen, from the Greek word for thread. Based on many observations of cells in various stages of division (Figure 1), Flemming correctly deduced the sequence of chromosome movements during mitosis , which would be confirmed decades later by microscopy of live dividing cells. With his characteristic attention to detail, Flemming also made the important observation that chromosomes split along their length during mitosis, and he correctly hypothesized that the split chromosomes were partitioned into different daughter cells at the end of mitosis.
Thus, Flemming recognized that chromosomal movement during mitosis offered a mechanism for the precise distribution of nuclear material during cell division. His work provided an invaluable description of the initial mechanisms underlying the process of cell division, and it helped paved the way for the discovery of hereditary mechanisms.
Theodor Boveri Links Chromosomes and Heredity
The end of the nineteenth century was marked by advancements in cytological techniques and microscopy. During this period, German embryologist Theodor Boveri took Flemming's findings to the next level by providing the first evidence that chromosomes of germ cell lineage provide continuity between generations. Boveri found evidence for this hypothesis through his research of early development in the roundworm species Ascaris megalocephala (now known as Parascaris equorum ). Ascaris embryos provided an excellent experimental model for Boveri's observations; this is because the large, clear cells of the Ascaris embryo have only two pairs of chromosomes, and the embryos develop distinct somatic cell and germ cell lineages during the first few cleavage divisions. Boveri was thus able to trace the fate of the chromosomes in individual cell lineages with great precision, and he made the surprising observation that the full complement of chromosomes was retained only in the Ascaris germ cell lineage, the source of future gametes . By contrast, the chromosomes in somatic cells, the source of all other adult tissues, underwent a curious process of fragmentation and elimination known as chromosome diminution. (Chromosome diminution does not occur in mammals.)
Boveri also recognized that chromosome number was reduced in the gametes. In Ascaris eggs, meiosis does not occur until fertilization is complete , so Boveri was able to observe the behavior of egg and sperm chromosomes following fertilization. He noted that Ascaris eggs retained only two chromosomes after the polar body formed, and that the normal number of four chromosomes was restored following fusion of the sperm and egg pronuclei. Boveri's work provided one of the first descriptions of meiosis.
Walter Sutton Finds Evidence for Mendel's Principles
Working independently near the dawn of the twentieth century, American graduate student Walter Sutton confirmed and expanded upon Boveri's observations using a superior cytological model. Sutton, a Kansas farm boy, had made the serendipitous discovery that it was possible to distinguish individual chromosomes in cells undergoing meiosis in the testes of the lubber grasshopper, Brachystola magna . Thus, in his classic paper published in 1902, Sutton described the configurations of individual chromosomes in cells at various stages of meiosis. Sutton was able to identify 11 pairs of chromosomes that could be distinguished by their sizes, as well as an accessory singleton that he correctly presumed to be a sex chromosome . Due to its apparent lack of a partner, this chromosome sorted into only half of the sperm cells, while the remaining half never received a copy. Though the accessory chromosome was unpaired, it still replicated and entered stages of mitosis in the same manner as all other chromosomes, which prompted Sutton to declare it a "true chromosome" and not simply an accessory.
Sutton postulated that all chromosomes have a stable structure, or "individuality," that is maintained between generations, and he used this property to follow the behavior of individual chromosomes through the various stages of meiosis, including synapsis . Most notably, Sutton recognized that his observations were consistent with the , whose findings had only recently been rediscovered. In fact, Sutton closed his 1902 paper with the statement, "I may finally call attention to the probability that the association of paternal and maternal chromosomes in pairs and their subsequent separation during the reducing division as indicated above may constitute the physical basis of the Mendelian law of heredity." With these words, Sutton articulated the chromosomal theory of inheritance.
Sutton subsequently went on to explain the basis for the ongoing variation among heritable traits. He noted that the position of each chromosome at the midline during metaphase was random, and that there was never a consistent maternal or paternal side of the cell division. Each chromosome was, therefore, independent of the others. When they separated into gametes, the set of chromosomes in each daughter cell could contain a mixture of the parental traits, but not necessarily the same mixture as that of other daughter cells. The newly discovered chromosomal independence during meiosis meant that the number of possible chromosomal combinations for each gamete could be calculated based on the number of chromosomes in the organism : specifically, there are 2 n possible combinations of chromosomes in gametes, with "n" representing the number of chromosomes in the gamete. Furthermore, considering all the possible pairings of one gamete with another, the variation in zygotes is (2 n ) 2 , which results in some fairly large numbers. Indeed, Sutton provided examples of the potential variation among hypothetical organisms with gamete chromosome numbers ranging from 1 to 19 (Table 1).He also correctly assumed that there was more than one trait present on each chromosome, so the actual total variation was even higher than any of those included in the table.

Thomas Hunt Morgan Experimentally Demonstrates Chromosome Theory
During the early years of the twentieth century, Thomas Hunt Morgan and his colleagues at Columbia University identified hundreds of Drosophila genes and made many pivotal discoveries about genetic transmission. Cytological examination showed that Drosophila possesses four pairs of chromosomes, including a pair of sex chromosomes . Female flies normally have two identical X chromosomes, whereas males have a single X chromosome and a smaller, gene-poor Y chromosome (Figure 2). Unlike humans, however, sex in fruit flies is determined by the number of X chromosomes, rather than by the presence of the Y chromosome.
One day, Morgan's associates discovered a male fly with unusual white eyes in their cultures. Breeding experiments quickly established that the white eye color was caused by a recessive mutation in the gene responsible for normal red eye color, and furthermore, that the gene was probably located on the X chromosome . The chromosome theory predicted that male flies would always display the eye color encoded on their single X chromosome, but that female flies would develop white eye color only when they inherited two mutant versions of the eye color gene. Thousands of matings confirmed this prediction, but on rare occasions, the group discovered "exceptional" white-eyed females among the progeny of a heterozygous female fly and a normal male, in apparent contradiction of the chromosome theory.
References and Recommended Reading
Benson, K. R. T. H. Morgan's resistance to the chromosome theory. Nature Reviews Genetics 2 , 469–474 (2001) doi:10.1038/35076532 ( link to article )
Bridges, C. B. Non-disjunction as proof of the chromosome theory of heredity. Genetics 1 , 1–52 (1916) ( link to article )
Brown, S. J. Entomological contributions to genetics: Studies on insect germ cells linked genes to chromosomes and chromosomes to Mendelian inheritance. Archives of Insect Biochemistry and Physiology 53 , 115–118 (2003)
Cannon, W. A. Cytological basis for the Mendelian laws. Bulletin of the Torrey Botanical Club 29 , 657–661 (1902)
---. Studies in plant hybrids: The spermatogenesis of hybrid cotton. Bulletin of the Torrey Botanical Club 30 , 133–172 (1903)
Mendel, G. Gregor Mendel's letters to Carl Nägeli. Genetics 35 , 1–29 (1950)
Sturtevant, A. H. A History of Genetics. (New York, Harper & Row, 1965)
Sutton, W. S. On the morphology of the chromosome group in Brachystola magna . Biological Bulletin 4 , 24–39 (1902)
---.The chromosomes in heredity. Biological Bulletin 4 , 231–251 (1903) ( link to article )
Wilson, E. B. Mendel's principles of heredity and the maturation of the germ cells. Science 16 , 991–993 (1902)
Winkelmann, A. Wilhelm von Waldeyer-Hartz (1836-1921): An anatomist who left his mark. Clinical Anatomy 20 , 231–234 (2007)
- Add Content to Group
Article History
Flag inappropriate.


Email your Friend

- | Lead Editor: Clare O'Connor

Within this Subject (43)
- Chromosome Analysis (10)
- Chromosome Number (4)
- Chromosome Structure (6)
- Chromosome Theory and Cell Division (5)
- Mutations and Alterations in Chromosomes (13)
- Sex Chromosomes (5)
Other Topic Rooms
- Gene Inheritance and Transmission
- Gene Expression and Regulation
- Nucleic Acid Structure and Function
- Chromosomes and Cytogenetics
- Evolutionary Genetics
- Population and Quantitative Genetics
- Genes and Disease
- Genetics and Society
- Cell Origins and Metabolism
- Proteins and Gene Expression
- Subcellular Compartments
- Cell Communication
- Cell Cycle and Cell Division

© 2014 Nature Education
- Press Room |
- Terms of Use |
- Privacy Notice |

Visual Browse

An official website of the United States government
The .gov means it’s official. Federal government websites often end in .gov or .mil. Before sharing sensitive information, make sure you’re on a federal government site.
The site is secure. The https:// ensures that you are connecting to the official website and that any information you provide is encrypted and transmitted securely.
- Publications
- Account settings
Preview improvements coming to the PMC website in October 2024. Learn More or Try it out now .
- Advanced Search
- Journal List
- HHS Author Manuscripts

Extrachromosomal DNA: An Emerging Hallmark in Human Cancer
1 Children’s Medical Center Research Institute, University of Texas Southwestern Medical Center, Dallas, Texas, USA
Vineet Bafna
2 Department of Computer Science and Engineering, University of California, San Diego, La Jolla, California, USA
Howard Y. Chang
3 Center for Personal Dynamic Regulomes and Howard Hughes Medical Institute, Stanford University, Stanford, California, USA
Paul S. Mischel
4 Department of Pathology, Stanford University School of Medicine, Stanford, California, USA
5 Chemistry, Engineering, and Medicine for Human Health (ChEM-H), Stanford University, Stanford, California, USA
Human genes are arranged on 23 pairs of chromosomes, but in cancer, tumor-promoting genes and regulatory elements can free themselves from chromosomes and relocate to circular, extrachromosomal pieces of DNA (ecDNA). ecDNA, because of its nonchromosomal inheritance, drives high-copy-number oncogene amplification and enables tumors to evolve their genomes rapidly. Furthermore, the circular ecDNA architecture fundamentally alters gene regulation and transcription, and the higher-order organization of ecDNA contributes to tumor pathogenesis. Consequently, patients whose cancers harbor ecDNA have significantly shorter survival. Although ecDNA was first observed more than 50 years ago, its critical importance has only recently come to light. In this review, we discuss the current state of understanding of how ecDNAs form and function as well as how they contribute to drug resistance and accelerated cancer evolution.
INTRODUCTION
The dynamic and rapid evolution of tumors presents one of the greatest challenges for developing more effective and durable treatments for cancer patients. Breakthroughs in genomic and epigenomic technologies, coupled with computational advances, have yielded unprecedented capacities to map the landscape of cancer, including within an individual patient. These capabilities have promised to usher in an era of precision cancer medicine. However, that promise has yet to be fulfilled for many patients with aggressive forms of cancer, because their tumor genomes seem to change rapidly in response to treatment at rates that are difficult to understand. These highly aggressive cancers are driven primarily by oncogene amplification, and recent research suggests that extrachromosomal DNA (ecDNA) may be the pivotal reason for this rapid tumor evolution. ecDNA is a remarkably effective way to cheat the system: It lacks centromeres and therefore is subject to non-Mendelian inheritance. Consequently, tumors with ecDNA evolve in unanticipated ways and at unexpected rates, because they change their genomes rapidly in response to changing selection pressures.
THE DISCOVERY AND REDISCOVERY OF EXTRACHROMOSOMAL DNA IN CANCER
The first series of tumor cases reporting the presence of ecDNA was documented in 1965 ( 1 , 2 ). At that time, it was given many names: double fragments of chromosome, double minutes, double bodies, minute chromatin bodies, and accessory chromatin. These early accounts already captured and presumed some of the crucial features of ecDNA, which are validated by modern biology:
- It was not found in matched blood cultures and was observed only in mitotic tumor cells.
- Its size varies.
- It may be subject to random segregation during mitosis.
- It could be circular, reminiscent of a ring chromosome.
Although the persistence of extrachromosomal particles in metaphases raised a debate regarding the presence of a functional centromere, subsequent research in 1978 showed that ecDNA does not have detectable centromeric activity ( 3 ). Notably, only 30% of ecDNAs occur as classic double-minute pairs ( 4 ); therefore, we use the term ecDNA, which describes both singlet ecDNA particles and double minutes.
In the late 1970s to 1980s, the content of ecDNA in cancer gradually became clear. The Schimke group ( 5 ) was the first to recognize that a stable and unstable increase in dihydrofolate reductase gene ( DHFR ) copy number contributed to murine cancer cells’ resistance to methotrexate. They soon realized that the unstable amplification of DHFR was associated with ecDNA, whereas the stable amplification was related to large chromosomes ( 6 – 9 ). Subsequently, more and more ecDNA species carrying important driver oncogenes were identified, including MYCN and MYC ecDNA, in various cancer types ( 10 , 11 ).
Although research into ecDNA in cancer has continued since its discovery, the literature has been relatively sparse in the past 50 years. With the development of contemporary genomic profiling technologies such as comparative genomic hybridization array and whole-genome sequencing (WGS), old-school cytogenetics seems to be left out in the cancer research field. However, the trade-off for high throughput and high sequence resolution is loss of spatial resolution, in which ecDNA information is buried underneath the rich body of data. In the past 20 years, many cancer genome sequencing projects identified hundreds of amplified oncogenic drivers. Yet, the question of where exactly these oncogenes are located was challenging to answer, as the sequencing data from a corrupted cancer genome were mapped to a normal reference genome to infer a DNA segment’s coordinate, assuming that every gene sits in a specific chromosomal location according to a traditional analysis pipeline.
In 2017, the first systematic investigation of ecDNA prevalence in human cancer was conducted on an NCI60 cell line panel, patient-derived cancer cell cultures, and clinical tumor tissues ( 4 ). This study combined WGS to call and analyze amplicon structure with microscopic examination of the metaphase cell in an unbiased way to directly visualize ecDNA, yielding a highly accurate ecDNA landscape in common cancer cell lines ( 4 ). Unlike a previous report based on the Mitelman Database of Chromosome Aberrations and Gene Fusions in Cancer stating that double minutes were present in only 1.4% of all cancers ( 12 ), the 2017 study revealed that ecDNA is surprisingly prevalent in cancer cells of various pathological types: Nearly 40% of tumor cell lines and nearly 90% of patient-derived brain tumor cultures were positive for ecDNA, which was almost undetectable in normal cells. The contradiction here is possibly due to differing definitions of ecDNA. The Mitelman database enumerates only the frequency of the double minutes, yet 70% of ecDNAs are in singlet form rather than in paired double minutes ( 4 ). Notably, there is no evidence showing that singlet ecDNA is biologically distinct from double minutes, and double minutes may simply represent the replicated form of singlet ecDNA found in early metaphase prior to segregation ( 13 , 14 ). More recently, a computational analysis of WGS data of more than 3,000 clinical cancer samples from The Cancer Genome Atlas (TCGA) and the International Cancer Genome Consortium (ICGC) cohorts again revealed a high prevalence of ecDNA, especially in the most aggressive and common tumor types—including brain, esophageal, ovarian, bladder, lung, breast, gastric, and cervical cancers—in which the presence of ecDNA is tightly associated with worse clinical outcomes ( 15 ).
Notably, although ecDNA is rarely observed in normal cells, both historical literature and modern research show evidence that ecDNA may exist in the normal tissue of cancer patients at an extremely low frequency, including in leukocytes and lymphocytes ( 4 , 15 – 18 ). However, whether the ecDNA represents contamination from circulating or metastatic tumor cells is not known, nor is the content of ecDNA in normal cells. In addition, ecDNA has been found in benign hyperplasia or precancerous cells at a low frequency, including in endometrial polyps and fibroblasts from patients with Bloom’s syndrome ( 19 , 20 ). However, whether the presence of ecDNA is associated with outcome in precancers remains to be explored.
MOLECULAR FEATURES AND FUNCTIONS OF EXTRACHROMOSOMAL DNA
Extrachromosomal dna is circular.
Since the discovery of ecDNA, the quest to reveal its physical shape has continued. Early ultrastructural studies using transmission electron microscopy uncovered some essential features of ecDNA. First, it is composed of nucleosomal chromatin and is organized into a certain degree of high-order fibers typical of the chromosome. Second, ecDNA appears to be circular, as no visible free ends were detected ( 21 – 23 ). Attempts to use polymerase chain reaction and mapping by restriction enzyme digestion of an MYCN ecDNA in a human neuroblastoma cell line also suggest a head-to-tail configuration in the nucleotide sequence ( 24 ). Scanning electron microscopy and atomic force microscopy were later used to reveal the ultrastructure. However, possibly due to resolution limitations, these images showed a spherical shape for ecDNA ( 14 , 25 , 26 ).
The most definitive proof establishing the circular shape was obtained in a study from late 2019 ( 27 ). This study combined the strength of DNA sequencing and high-resolution imaging. First, short-read WGS with amplicon architecture analysis revealed a circular configuration of several DNA segments joined together with breakpoints in between. Second, long-range optical mapping, revealed a continuous contig that spans across all breakpoints of ecDNA, further supporting the circular shape of the ecDNA particle. Third, all ultrastructural imaging data, including scanning and transmission electron microscopy as well as 3D structured illumination microscopy, showed a ring shape, indicating that ecDNA is unequivocally circular. The confirmation that ecDNA is circular, coupled with techniques to map its sequence content, has made it possible to produce individualized maps of ecDNA particles, including in individual tumor samples ( Figure 1 ).

A circular map of circular extrachromosomal DNA (ecDNA). Current evidence unequivocally shows that ecDNA is circular. However, traditional genome browsers still use linear maps, which cannot show the true nature of ecDNA. ( a ) For ecDNA with a simple structure (e.g., EGFR ecDNA in GBM39 cells), a linear map may still be useful. ( b ) However, ecDNA with complicated rearrangements (e.g., MYC ecDNA in COLO320DM cells) is difficult to visualize with a linear map, while a circular map helps disambiguate the orders and orientations of rearranged genomic segments, including material that is duplicated within an ecDNA. Abbreviation: FISH, fluorescence in situ hybridization. Linear and circular maps were created on the basis of publicly deposited whole-genome sequencing data ( 27 ).
Extrachromosomal DNA Drives Massive Oncogene Expression Due to High Copy Number and Decompacted Chromatin
Circular ecDNA serves as a template to direct gene transcription, which is revealed by allele-specific RNA-sequencing analysis ( 27 ). More importantly, genes encoded on ecDNA, especially oncogenes, are usually highly expressed; the abundance of some transcripts can reach the top 1% of the tumor’s transcriptome, which indicates that ecDNA may play a critical role in amplifying oncogenic signaling in cancer ( 27 ).
ecDNA promotes high oncogene expression through at least two mechanisms. First, ecDNA can be detected at high copy number levels that are not observed with other forms of gene amplification ( 27 ). It is not uncommon to see dozens to more than a hundred ecDNA particles in a cancer cell. Compared with chromosomal amplification, the copy number of ecDNA is usually higher, sometimes even higher than that observed in breakage-fusion-bridge (BFB) amplicons ( 15 , 27 ). The ability to acquire such a high copy number may relate to the unequal segregation of ecDNA during mitosis, which is discussed in the section titled Extrachromosomal DNA Mediates Rapid Tumor Evolution, below.
Yet, the high copy number explains only part of the mechanism. Recent studies have demonstrated that ecDNA transcribes higher levels of oncogenes compared with copy-number-matched chromosomal DNA ( 15 , 27 ). The human genome is segmented into hierarchical chromatin structures with differential accessibility and organizes transcriptional activity into individual compartments, whose configuration is tightly linked to the chromatin’s epigenetic landscape ( 28 ). Quantitative assessments of ecDNA accessibility by ATAC-seq (assay for transposase-accessible chromatin with high-throughput sequencing) and ATAC-see (assay of transposase-accessible chromatin with visualization) have revealed that ecDNA contains the most accessible chromatin in the cancer genome and lacks the higher-order compaction typical of heterochromatin, thereby allowing higher transcriptional activity ( 15 , 27 ).
Circular Extrachromosomal DNA Forms a Novel Cis -Regulatory Circuit
DNA also codes information in its shape. Once circularized, DNA segments of ecDNA form a new chromatin domain distinct from their chromosomal counterparts. The most significant outcome is that DNA elements farther away are brought into proximity due to DNA circularization, forming novel cis -regulatory circuits that are not possible in chromosomal DNA ( 27 ). Therefore, ecDNA is a powerful enhancer hijacking vector in cancer.
There are two enhancer hijacking models in ecDNA: local and distal. In the local hijacking model, a cluster of enhancers, up to a few megabases (the upper limit size of an ecDNA) distal to an oncogene, are brought into proximity by DNA circularization. The enhancer cluster can be isolated from the oncogene by an insulator in the chromosomal DNA. However, once this enhancer cluster is coamplified with the oncogene and circularized as ecDNA, the enhancers can trespass the insulator and become accessible to the oncogene ( Figure 2a ). In glioblastoma, the oncogenic driver gene EGFR often coamplifies with the upstream enhancer and forms ecDNA, creating new enhancer–oncogene contacts and contributing to cancer cell growth ( 29 ). Local enhancer hijacking is also found in neuroblastoma ecDNA containing the oncogene MYCN ( 30 ).

Extrachromosomal DNA (ecDNA) acts in cis and in trans . ( a ) ecDNA is a vector of enhancer hijacking. By incorporating an enhancer from an adjacent topologically associating domain into a circle, or from a distal region such as a different chromosome by chimeric circularization, the oncogene encoded on ecDNA can access a variety of enhancers through cis -interactions that are not possible in chromosomes. ( b ) ecDNA can act in trans with other DNA, including ecDNA–chromosomal DNA interaction and ecDNA–ecDNA interaction.
The distal enhancer hijacking model refers to the mechanism by which enhancer segments and oncogene segments that are physically far apart are joined together to form an ecDNA circle. These DNA segments could originate from the same chromosome, or even different chromosomes. Therefore, this model enables ecDNA to create complicated and heterogeneous domains to regulate oncogene expression that expand the possibilities for oncogene hijacking ( Figure 2a ). ecDNA-directed distal enhancer hijacking is common in neuroblastoma and is associated with worse clinical outcomes ( 30 , 31 ).
Extrachromosomal DNA Is a Mobile Trans -Acting Element
Eukaryotic DNA in the nucleus is folded into chromosomes and subsequently organized into chromosomal territories, where each chromosome occupies a specific space inside the nucleus. This spatial organization is critical to the stability and physiological function of the chromosome, including acting as an architecture for chromatin interaction within a chromosome, thereby limiting interchromosomal contact within specific regions of the chromosome exteriors ( 32 , 33 ). Although it is unclear whether ecDNA in cancer is also well organized spatially, microscopic imaging has shown that ecDNA is scattered throughout the nucleus. Furthermore, this observation suggests that, due to its high copy number and small size, ecDNA is mobile and may freely interact with other DNA.
Recent research mapping ecDNA–chromosomal DNA interactions suggests that ecDNA can act as a mobile trans -acting element ( Figure 2b ). The interaction of ecDNA with chromosomal DNA appears to be genome-wide. Furthermore, hundreds of trans -interaction sites have been identified by RNA polymerase II ChIA-PET (chromatin interaction analysis with paired-end tag sequencing), showing that interactions produce an elevated transcriptional potential. More importantly, ecDNA is enriched for enhancers, which interact with chromosomal DNA more frequently. These findings are the basis of a new concept, that ecDNA can function as a mobile enhancer to regulate chromosomal gene expression, including that of oncogenes ( 34 ).
As ecDNA is highly amplified and mobile, it is not difficult to imagine that one ecDNA particle can interact with another ( Figure 2b ). A recent study ( 35 ) demonstrates that approximately 10–100 ecDNA particles can cluster together to form an interaction hub and promote oncogene expression. For example, a subspecies of MYC-PVT1 ecDNA lacking enhancers can access another ecDNA subspecies carrying enhancers within the ecDNA hub.
Extrachromosomal DNA as a Source of Somatic Rearrangement
Historically, ecDNA has been thought of as an unstable form of gene amplification that is sensitive to different microenvironments with different selection pressures. The modern concept regarding instability can expanded to structural and spatial dynamics, in which an ecDNA particle can change in its sequence and localization during cancer evolution ( 7 , 9 , 36 ).
Accumulating evidence suggests that the complexity of ecDNA can increase during tumor evolution. In the late 1980s, an episome model was proposed wherein ecDNA can arise from small, submicroscopic extrachromosomal particles (episomes) that eventually enlarge into microscopically visible ecDNA ( 36 ). Although not all ecDNAs observed to date are derived from episome aggregation and fusion ( 37 , 38 ), amplicon structural analyses suggest that DNA segments can be added into or deleted from an existing ecDNA, creating new ecDNA species in cancer ( 39 , 40 ). The molecular mechanism governing ecDNA rearrangement is unclear. However, because ecDNA widely interacts with chromosomal DNA and naturally forms ecDNA hubs ( 34 , 35 ), it is possible that these ecDNA interaction foci may be functional units for ecDNA rearrangement. Further research is needed to complete the missing pieces of this puzzle.
More importantly, ecDNA can reintegrate into the chromosome. Building on earlier observations made using Southern blots ( 9 , 36 ), modern sequencing technologies and bioinformatic analyses have revealed several important aspects of the reintegration:
- Cellular stress, including drug treatment and DNA double-strand breaks, can drive reintegration ( 4 , 41 , 42 ).
- Several ecDNA particles can aggregate together and then reintegrate into the chromosome, echoing the episome model ( 4 , 36 ).
- The integrated element can disrupt the integrity of the chromosomal genes in the integration site. For example, in neuroblastoma, ecDNA reintegration can destroy the gene body of the tumor suppressor gene DCLK1 , leading to decreased expression, and enhance the expression of TERT , potentially by enhancer hijacking ( 31 ).
In addition, the birth of an ecDNA may enable gene fusion due to chimeric circularization of DNA segments from one or more chromosomes ( 31 , 38 , 39 ). Pan-cancer analyses show that transcript fusions occur in ecDNA with a fivefold frequency compared with their occurrence in other types of amplification ( 15 ). However, further studies are needed to understand the functional consequence of ecDNA-driven gene fusion in cancer.
EXTRACHROMOSOMAL DNA MEDIATES RAPID TUMOR EVOLUTION
The first functional impact of ecDNA discovered is probably how it mediates drug resistance, thanks to a series of studies by the Schimke group during the late 1970s and early 1980s. Schimke and colleagues first documented that DHFR is selectively amplified in methotrexate-resistant cell lines, which existed in stable and unstable forms ( 5 ). Subsequent research linked ecDNA to unstable drug resistance, which was rendered stable by reintegration of ecDNA particles into the chromosome, creating homogeneous staining regions (HSRs) ( 6 – 9 ). More recently, BRAF-V600E mutant gene amplification as ecDNA was found to govern ERK inhibitor resistance in PDX models ( 43 ).
The response of EGFR ecDNA–carrying glioblastoma cells to EGFR-targeted therapy ( 41 ) presents a stark contrast to the above examples. Drug treatment using the kinase inhibitor erlotinib led to a rapid decline in EFGR ecDNA copy number, resulting in resistance. Some of the ecDNA even reintegrated into the chromosome to form an HSR-like structure with low gene activity. With the loss of the drug target, the cancer cells no longer responded to the treatment. Upon drug removal, the EGFR ecDNA rapidly reemerged and drove tumor growth ( 41 ). All of these studies highlight the powerful and disparate mechanisms by which ecDNA causes therapeutic resistance through dynamic copy number changes.
ecDNAs are acentric, as revealed by imaging and sequencing ( 3 , 4 ). Notably, lack of a functional centromere does not substantially affect ecDNA’s segregation efficiency, because ecDNA can hitchhike with chromosomes to segregate into daughter cells ( 44 , 45 ). Nevertheless, there is no mechanism to ensure equal ecDNA segregation during mitosis. The segregation direction of ecDNA is a coin toss, which leads to a binomial distribution of ecDNA in daughter cells, freeing ecDNA from the constraints of Mendelian inheritance ( 46 , 47 ).
The random segregation of ecDNA drives rapid tumor evolution by at least two mechanisms. First, it allows a portion of cancer cells to acquire high oncogene copy numbers rapidly, and second, it creates an enormous pool of genetically heterogeneous cancer cells to promptly adapt to the ever-changing microenvironment and selection pressures ( 4 ). It is also possible that the unique cis -interactions within an ecDNA particle ( 27 , 29 – 31 ) and the prevalent ecDNA–ecDNA and ecDNA–chromosomal DNA trans -interactions may contribute to accelerated tumor evolution ( 34 , 35 ). Furthermore, potential ongoing mutagenesis of ecDNA may also have an effect on tumor evolution.
If we imagine that there are three identical ecDNA particles in a mother cell that become six particles after DNA replication in S phase, upon mitosis the ecDNA particles will randomly segregate into two daughter cells, which may receive from zero to six particles. Therefore, ecDNA can create genetic heterogeneity in just one cell cycle ( Figure 3 ). If a higher oncogene copy number favors advantaged cell growth, the daughter cells with more ecDNA will expand more rapidly and will continue to increase oncogene copy number until hitting some threshold that limits cellular fitness. This explains why methotrexate and ERK inhibitor treatment increases the copy number of DFHR and BRAF-V600E mutant genes, respectively. Similarly, if selection pressure opposes high oncogene copy number, such as EGFR kinase targeting in glioblastoma, a tumor type rarely containing point mutations in the EGFR kinase domain, the EGFR ecDNA copy number declines rapidly through negative selection, and EGFR ecDNA may reintegrate into a chromosome and deactivate its transcriptional activity through an unknown mechanism ( 41 ).

Unequal segregation of extrachromosomal DNA (ecDNA) drives rapid tumor evolution. Acentric ecDNA segregates unequally to two daughter cells during cell division. Therefore, each cell division generates genetic heterogeneity in the cancer population, allowing a portion of cancer cells to gain oncogene copy number rapidly. Furthermore, this process creates a pool of genetically heterogeneous cancer cells for microenvironmental selection, increasing cancer fitness and promoting rapid cancer evolution.
The foundational principles of Darwinian evolution are variation, selection, and identity by descent. However, the impact of nonchromosomal oncogene inheritance—random identity by descent—is not well understood. Recent research integrating mathematical modeling, unbiased image analysis, CRISPR-based ecDNA tagging, and live-cell imaging has revealed a set of basic “rules” for how random ecDNA inheritance drives oncogene copy number and distribution, resulting in extensive intratumoral ecDNA copy number heterogeneity and rapid adaptation to metabolic stress and targeted cancer treatments ( 48 ).
Segregation of ecDNA in cancer cells is reminiscent of plasmid-directed hereditary mechanisms in bacteria, which are potent drivers of the genetic variation that underlies the rapid appearance of cells with a selective advantage ( 49 ). In cancer, ecDNA may be even more powerful because multiple species of ecDNA can coexist in one cancer cell and even interact with other DNA, adding another layer of complexity to the random segregation mechanism ( 27 , 34 , 35 ). With the increase of ecDNA species in the cancer cell population, the heterogeneity increases more dramatically, facilitating even faster tumor evolution.
THE ORIGIN OF EXTRACHROMOSOMAL DNA
How does ecDNA originate? There appear to be multiple paths toward ecDNA formation, each of which involves some type of DNA damage, usually occurring in the context of tumor suppressor losses. However, the types of DNA damage and the factors triggering them are diverse.
Chromothripsis
The best-known origin of ecDNA is chromothripsis, in which a chromosome is broken into small pieces due to catastrophic DNA damage and undergoes massive DNA rearrangement by religation, acting as a powerful driver of karyotype evolution in cancer ( 50 ). In some cases, a few shattered DNA fragments can be stitched back together as circular ecDNA ( Figure 4a ). Early evidence has shown that chromosome missegregation can lead to micronucleus formation, where chromothripsis occurs and generates megabase-scale circular chromosomes based on sequencing ( 51 ). A recent study using a model system that selectively induces lagging Y chromosome formation has also demonstrated that the chromothriptic Y chromosome can give rise to ecDNA particles, along with many structural aberrations ( 52 ). More importantly, multiple studies have documented that the fragments that form circular amplicons are missing from the chromothripsis-derived chromosome, further supporting the idea that the reassembly of DNA fragments can generate ecDNA ( 51 , 53 – 55 ). In addition, continuous BFB cycles, which can generate >100-Mb chromosome arms, are also responsible for ecDNA formation, as the abnormal chromosome is prone to becoming trapped within interphase bridges and shattered, generating ecDNA by chromothripsis ( 42 ).

Potential pathogenesis pathways of extrachromosomal DNA (ecDNA). ( a ) Religation of shattered DNA segments from chromothripsis can form ecDNA. ( b ) Two DNA double-strand breaks in one arm of a chromosome can create ecDNA by religation of the excised segment, leaving a scarred chromosome. ( c ) If mild DNA damage occurs between two replication foci, the chromosome may be repaired through a homologous recombination mechanism while generating an ecDNA particle. ( d ) Speculative model for ecDNA generation through fork stalling and template switching. In this model, a DNA lesion occurs in the template strand, stalling the lagging strand in DNA replication. The lagging strand can disengage from the current template and invade the adjacent replication fork through microhomology to continue DNA synthesis. Strand disengagement and invasion can occur over multiple rounds until the strand returns to the original template. Although the mechanism is unclear, this process may generate ecDNA through DNA repair.
However, on the basis of DNA copy number and structural variation analysis in the pan-cancer data set, although half of the ecDNA-positive cases have chromothripsis in any part of the genome, only ~36% of the DNA segments forming ecDNAs show a chromothripsis signature ( 15 ). These data show that a substantial fraction of ecDNAs originate through a mechanism other than catastrophic chromosome damage and religation.
Mild DNA Damage and Religation
In theory, if a piece of the linear double-stranded DNA segment is ligated head-to-tail, circular DNA forms. Could this actually happen inside the cell nucleus? A recent study using CRISPR to fragment and circularize a fluorescent biosensor (CRISPR-C) provides an answer. This approach generates endogenous extrachromosomal circular DNAs (eccDNAs) in sizes ranging from 10 2 to 10 5 bp, and even a 47.4-Mb ring chromosome, by nonhomologous end-joining and blunt end-joining repair processes ( 56 ). More importantly, although the DNA circles generated by CRISPR-C gradually disappear, the kinetics is slower than in the theoretical dilution model, indicating that a replication mechanism is involved ( 56 ). This study suggests that a DNA double-strand break creating a free DNA segment is sufficient to generate eccDNA, which replicates during the cell cycle ( Figure 4b ). On the basis of these findings, we hypothesize that if the DNA circle contains an oncogene that enhances cellular fitness, the cell with the DNA circle will be preserved and gain a fitness advantage. Due to the unequal segregation that creates a pool of cells with various copy numbers of circles, a population with an optimal range of DNA copy numbers will rapidly emerge, ultimately becoming cancer if other regulators such as tumor suppressors are disabled.
Studies focusing on ecDNAs and their chromosomal origins have shed light on the mild DNA damage model. In the 1980s, when comprehensive genomic profiling was not available, Wahl and colleagues ( 36 ) used Southern blotting to study the episome dynamic in an artificial system. Their results suggested that ecDNA formation is associated with the loss of chromosomal sequence. More recently, fluorescence in situ hybridization (FISH) in a leukemia case revealed that the presence of MYC ecDNA is accompanied by the deletion of one MYC locus on chromosome 8, suggesting that MYC ecDNA arises from the lost copy of chromosomal MYC ( 57 ). Furthermore, chromosome walking and microarray analyses indicate that, in some glioma cases, the chromosomal origins of ecDNAs do not have the extensive DNA copy number oscillations that are typical of chromothripsis ( 58 ). More importantly, the chromosome of origin may or may not have a deleted locus corresponding to the ecDNA segment ( 58 , 59 ). These observations raise two potential ecDNA pathogenesis pathways:
- One or a few DNA segments are excised from the same chromosome or different chromosomes and fused to form ecDNA, leaving scars on the chromosome of origin ( Figure 4b ).
- A double-stranded DNA segment is excised between two replication forks and forms ecDNA, and the chromosomal scar is healed by rereplication ( 59 ) ( Figure 4c ).
Interestingly, one study suggests that a V(D)J-like recombination mechanism may be involved in ecDNA formation, as some of the ecDNA junctions were found to have ectopic recombination signal sequences ( 58 ).
Fork Stalling and Template Switching
A homology analysis at the ecDNA breakpoints suggests that fork stalling and template switching during DNA replication is another possible origin of ecDNA ( 60 ). In this model, a DNA replication fork stalls at the lesion, where the lagging strand disengages from the current template, invades and anneals to an active adjacent replication fork by microhomology, and continues to synthesize nascent DNA. Strand invasion and resynthesis could happen multiple times before the strand eventually returns to its original template. Therefore, the nascent DNA synthesized during template switching is not entirely complementary to the original template, resulting in a single-stranded DNA bulge in either strand ( 61 ). Although this model has not yet been experimentally proven, the single-stranded DNA could be a source of ecDNA ( Figure 4d ).
Genome Instability
Emerging studies have begun to determine which genetic background is permissive for ecDNA pathogenesis. One hypothesis is that loss of genomic guardians, including cell cycle checkpoint and DNA repair pathways, is essential to initiate ecDNA formation. In mouse mammary tumors harboring Brca1 and Trp53 deletions, the oncogene Met is frequently amplified as ecDNA in vivo. Interestingly, these Met ecDNAs are gradually lost after a few passages in culture, suggesting that the in vivo microenvironment is required for ecDNA maintenance ( 62 ). Mouse NIH-3T3 cells transformed through overexpression of the cell cycle promoter Sertad1 (formerly called Sei-1 ) can also generate Met ecDNAs in vivo that gradually disappear during in vitro culture ( 63 , 64 ).
Functional integrity of SIRT1 is essential to prevent DNA breakage upon replication stress. Cells with compromised SIRT1 , including loss of expression and inability to phosphorylate its T530 site, are prone to generate extrachromosomal elements. Treatment with aphidicolin, an agent that induces replication stress, can further increase the number of extrachromosomal elements that appear in cells with compromised SIRT1 ( 65 ). However, the physical shape, size, content, and stability of these extrachromosomal elements have not yet been characterized. Therefore, whether these extrachromosomal particles resemble functional ecDNA in cancer or are transient products of DNA breakage remains unclear.
The sequence and structure of ecDNA can continue to evolve after it is formed. Historically, the episome model proposed that large, microscopically visible ecDNA is derived from small, submicroscopic circular episomes, which can gradually enlarge in cultured cells ( 36 ). Although later research showed that episomal evolution is not essential ( 37 ), it does not rule out the possibility that the structure and content of ecDNA are capable of changing over time. Modern structural analyses using WGS found, in a specimen from a patient with esophageal carcinoma, that a large KRAS ecDNA was composed of two KRAS -containing DNA segments assembled in a mirror-repeat fashion, suggesting that two smaller ecDNAs merged together ( 27 ). Continuous evolution of ecDNA structures has been detected by tracking amplicon structural variation during tumor progression. For example, in a glioblastoma case, new ecDNA species emerged at tumor relapse that were generated by integration of new oncogene-containing segments into the ecDNA found at diagnosis ( 66 ).
MAINTAINING EXTRACHROMOSOMAL DNA IN CANCER CELLS
ecDNA imposes a barrier to traditional oncogene targeted therapy due to its heterogeneity and dynamic ability to rapidly change tumor genomes. Therefore, targeting the mechanisms that regulate ecDNA itself may be necessary to yield benefit. To this end, we need to understand ( a ) the molecular mechanisms of ecDNA formation (discussed above), so that we can prevent the appearance of ecDNA up front during therapy, and ( b ) the maintenance mechanisms of ecDNA, so that we can tackle these pathways to disarm ecDNA in cancer cells.
To date, it is still not very clear what cellular contexts are required to maintain ecDNA in cancer cells. As mentioned above, multiple studies have shown that primary isolated cancer cells will lose ecDNA over time during culture but tend to retain ecDNA if maintained as xenografts, suggesting that the in vivo microenvironment is critical in producing selection pressure favoring ecDNA ( 62 – 64 , 67 – 70 ). Even ecDNAs that are generated in vitro, such as methotrexate-driven DHFR ecDNAs, could disappear without selection pressure ( 5 , 8 , 71 ). However, many established cancer cell lines under regular culturing conditions in vitro also stably host ecDNA ( 4 ), presumably because in vitro culturing environments maintain selection pressure. But what exactly are these selection pressures? Do they represent specific microenvironments interacting with specific metabolic programs, genetic contexts, and cellular signals?
Hydroxyurea treatment and radiation, both of which damage DNA, can lower ecDNA levels in some contexts ( 72 – 78 ), but the mechanism of damage has not been established. Its relative specificity for ecDNA and its potential differential efficacy against ecDNA-driven cancers remain open questions. One consistent finding is that, once damaged, ecDNA particles often aggregate and subsequently form micronuclei ( 76 – 78 ). It is still unclear why aggregation happens in this situation and whether it is linked to ecDNA associated with homologous repairing templates, though ecDNA hubs form naturally and participate in cooperative transcriptional regulation ( 35 ). Interestingly, silencing the homologous recombination repair gene BRCA1 was reported to decrease the DFHR ecDNA number in one methotrexate-resistant cancer cell line ( 79 ).
Several other studies have attempted to address what genetic background and signals are required for ecDNA maintenance. One report suggests that SIRT1 is responsible for stabilizing extrachromosomal particles, as knocking out SIRT1 results in the loss of these particles ( 80 ). However, this study was conducted with a transfected episomal plasmid in a COLO320DM cell line that already carries native ecDNAs ( 27 ). While the plasmid episomes were lost in the SIRT1 -null background, it will be necessary to investigate whether SIRT1 is required to maintain endogenous ecDNA. Another study showed that inhibiting Met signaling reduced ecDNA numbers in Sertad1 -transformed NIH-3T3 cells ( 64 ). However, because these ecDNAs carry Met , the observed phenomenon may also be explained by selection pressure against Met . Specifically, under therapy, the cell population with a high Met copy number that is addicted to Met signaling may be eliminated by Met inhibition, regardless of the form of Met amplification. Therefore, Met signaling may not represent a universal mechanism underlying ecDNA maintenance.
CURRENT TOOLBOX FOR EXTRACHROMOSOMAL DNA RESEARCH
Imaging-based approaches.
Seeing is believing. Microscopic examination of cells at metaphase remains the gold standard to identify the existence of ecDNA. The classic protocol involves enriching the cell population at metaphase by drug treatment (e.g., with colcemid) or by mitotic shake-off, swelling mitotic cells with hypotonic buffer, fixing with 3:1 methanol acetic acid fixative, dropping the cells onto a humidified slide, and finally staining with DNA dye. With downstream FISH, this approach can unequivocally determine whether a gene is amplified on ecDNA.
Algorithms and software have recently been developed to detect and quantify the number of ecDNAs across large cell populations. ecDetect uses computer vision–based methods to identify and quantify ecDNA in 4’,6-diamidino-2-phenylindole (DAPI)-stained images in a semiautomated fashion. It was designed to minimize false positives and has very high precision but also somewhat lower sensitivity than visual counting, leading to an undercounting of ecDNA, particularly ecDNA particles close to chromosomes ( 4 ). A more recent method, ecSeg, utilizes a deep neural network approach to improve the sensitivity of DAPI-stained ecDNA identification in metaphase cells and allows for the integration of FISH signals ( 81 ). While the models for these methods were trained largely using an image set generated by a single laboratory using established cancer cell lines, the availability of additional training data sets should provide the community with a robust analysis tool for microscopic images generated by different labs from different sample sources.
However, imaging-based technology has limitations:
- Metaphase cell preparation is not always feasible because it requires viable, cultured cells.
- Some ecDNAs may be too small to visualize with DNA dyes alone, though FISH can significantly enhance the sensitivity of visual detection.
- Without prior sequencing or other profiling information to determine the amplified region, it is not possible to perform FISH.
- Throughput is low.
Therefore, universal, unbiased, and high-throughput solutions are urgently needed.
Sequencing-Based Approaches
Sequencing and computational technologies have become popular options to determine the structure, content, copy number, and diversity of ecDNA in cancer. Importantly, the development of sequence-based methods is critical for analyzing ecDNA scope, scale, and sequence content from publicly available cancer genome databases and tumors excised from patients who will be studied in clinical trials, a context in which live tumor cells are often unavailable.
The first strategy is to directly sequence the whole genome and look for discordant reads that support a circular architecture. In this scenario, short-read WGS is possibly the most cost-effective and widely used way to detect ecDNA genome-wide. Generally, 10× coverage of short-read WGS is sufficient to identify ecDNA. Even shallow sequencing with coverage down to 1× is feasible, as the genomic segments composing ecDNAs are amplified to very high levels, often 10–100× ( 4 ). AmpliconArchitect software, used with the preprocessing script PrepareAA and a postprocessing classification tool called AmpliconClassifier, is a powerful tool for analysis of amplicons and extraction of ecDNA information from short-read WGS data. AmpliconArchitect explores an amplified region using discordant read mapping to reconstruct an amplicon graph that describes the architecture (the order and orientation of amplified genomic segments) of ecDNA ( 82 ). ecDNA prediction using AmpliconArchitect was recently validated by comparisons to metaphase FISH in multiple cancer cell lines, including NCI60, with a positive predictive value of 85% and a sensitivity of 83% ( 4 , 15 , 27 ). The high accuracy of ecDNA prediction led to an exploration of the ecDNA landscape using short-read WGS data from more than 3,000 cancer patients in the TCGA and ICGC cohorts ( 15 ). Notably, results from WGS with AmpliconArchitect are almost 100% concordant with those from Circle-seq, a library enrichment method optimized for circular DNA detection, further supporting the fidelity of this approach.
However, although short-read WGS has the highest base-pair resolution, the read length is usually 100–200 bp, limiting the capacity to resolve the complicated structural rearrangement of an amplicon of the size of ecDNA, which ranges from hundreds of kilobases to several megabases. Technologies such as nanopore long-read sequencing and optical mapping can mitigate this limitation and are proven approaches to resolving ecDNA architecture ( 27 , 30 ). Using a newly developed algorithm, AmpliconReconstructor, recent research has demonstrated that the combination of short-read WGS and optical mapping provides a high-fidelity, cost-effective way to resolve complicated amplicon architectures ( 83 ).
Investigators have proposed alternative direct sequencing and analyzing tools, such as ATAC-seq with Circle_finder software, that can identify both the small circular DNA and the large, oncogene-containing ecDNA in cancer ( 84 ). Additionally, the genomic fragments sequenced after enrichment often do not contain paired edges connecting different junctions, resulting in a loss of information as to whether different genomic segments are part of the same or different ecDNA. A side-by-side benchmarking of WGS and ATAC-seq with the corresponding analysis software will be necessary for the field to select the optimal tool to identify ecDNA by sequencing.
The second strategy used to search for ecDNA is to enrich for circular DNA before sequencing. Traditionally, circular DNA isolation involves cesium chloride–ethidium bromide density gradient centrifugation and 2D gel electrophoresis ( 85 , 86 ). Column- or magnetic bead–based methods to isolate high-molecular-weight DNA, followed by removal of chromosomal DNA by exonuclease, have recently become popular. One of the representative methods is Circle-seq, which has been used to characterize small, nonamplified, usually non-gene-containing eccDNAs ( 87 , 88 ) as well as large, highly amplified, oncogene-enriched circular ecDNAs in cancer. Circle-seq has been applied to neuroblastoma, revealing the structure of crucial oncogenes such as MYCN that are frequently amplified as ecDNA in this neoplasm ( 31 ).
Compared with direct sequencing approaches, enrichment strategies theoretically improve the specificity of identifying circular ecDNA by eliminating amplicons generated by BFB or found in homogeneously staining regions. However, the key to success is to protect the integrity of high-molecular-weight ecDNA from shearing before exonuclease digestion, which is still technically challenging, especially for large ecDNAs that are several megabases long. Technologies including automatic liquid handling and slow pipetting may improve sample integrity and consistency during preparation. In addition, most enrichment protocols involve rolling-circle amplification of ecDNA before sequencing. Although the fidelity of the polymerase Phi29 is high, amplification bias and the potential introduction of mutations during amplification of ecDNA remain to be evaluated.
CONCLUDING REMARKS
The 23 pairs of chromosomes are the blueprint of human life. They are profoundly altered in cancer, driving uncontrolled growth. The discovery and rediscovery of ecDNA have shed new light on how cancers can hack genetic codes and rapidly change their genomes by changing the location and the topology of organization of their DNA, in addition to revealing some of the other well-known mechanisms involving changing their sequence and abundance.
ecDNA imposes a barrier to precision oncology and targeted cancer therapy. Future studies will be needed to better understand the molecular pathogenesis mechanisms of ecDNA formation, function, maintenance, and vulnerability, as well as their interaction with the microenvironment, including the immune system, to overcome these barriers and develop more effective treatments for patients with some of the most aggressive cancers. Although ecDNA was first observed more than 50 years ago, this prescient work on its potential importance and its scale, scope, and impact was not well understood until recently. Powerful new integrative molecular approaches have shown us that ecDNAs are present in nearly half of all human cancer types and likely in at least a quarter of all cancer patients. They have taught us that ecDNA is, indeed, one of the most urgent problems facing patients with cancer. Our new knowledge of ecDNA has catalyzed a shift in our understanding of gene amplification in cancer, lending a powerful insight into the accelerated evolutionary trajectory of some tumors that have surprised and foiled so many modern treatment strategies. The presence of ecDNA also makes us recognize, once again, that DNA conveys information not only in its sequence but also in its shape, and that we will need to come to grips with the fact that altered DNA topology is a central feature of cancer pathogenesis. Finally, ecDNA challenges the successful implementation of targeted cancer therapies, clearly indicating that it is a problem worthy of its nomination as a Cancer Grand Challenge ( 89 ). We look forward to the collective engagement of the field to develop new fundamental understandings of ecDNA in human cancer, and to develop and deploy new treatments for patients with some of the most aggressive forms of cancer.
ACKNOWLEDGMENTS
S.W. is a scholar of and is supported by the Cancer Prevention and Research Institute of Texas (RR210034). H.Y.C. is an investigator of the Howard Hughes Medical Institute and supported by R35-CA209919. V.B. is supported by U24CA264379 and R01GM114362. P.S.M. is supported by RO1-CA238249.
DISCLOSURE STATEMENT
V.B. is a cofounder, consultant, and Scientific Advisory Board member of and has equity interest in Boundless Bio, Inc. (BB) and Abterra Bio, Inc. The terms of this arrangement have been reviewed and approved by the University of California, San Diego, in accordance with its conflict-of-interest policies. H.Y.C. is a cofounder of Accent Therapeutics and BB and an advisor for 10× Genomics, Arsenal Biosciences, and Spring Discovery. P.S.M. is a cofounder of BB. He has equity in the company and serves as the chair of its Scientific Advisory Board, for which he is compensated.
LITERATURE CITED
If you're seeing this message, it means we're having trouble loading external resources on our website.
If you're behind a web filter, please make sure that the domains *.kastatic.org and *.kasandbox.org are unblocked.
To log in and use all the features of Khan Academy, please enable JavaScript in your browser.
Course: MCAT > Unit 6
Chromosomal inheritance questions.
- MCAT training passage: A family history of Marfan syndrome
- Evidence that DNA is genetic material 1
- Evidence that DNA is genetic material 2
- Sex-linked traits
- Worked example: Punnett squares
- Genetic recombination
- Gene mapping
- Extranuclear inheritance 1
- Extranuclear inheritance 2

- (Choice A) Biomolecular composition of chromosomes A Biomolecular composition of chromosomes
- (Choice B) Transformation using heat-inactivated bacteria B Transformation using heat-inactivated bacteria
- (Choice C) Presence of DNA in all cells C Presence of DNA in all cells
- (Choice D) Mechanism of semi-conservative DNA replication D Mechanism of semi-conservative DNA replication
- MCQ on Chromosomal Basis of Inheritance
MCQs on Chromosomal Basis of Inheritance
1. The term chromosome was coined by ___________.
(c) Waldeyer
(d) Hoffmeister
Sol: (c) Waldeyer.
2. Chromosomes found in the salivary gland of Drosophila is___________.
(a) Polytene
(b) Lampbrush
(c) Supernumerary
(d) B-chromosomes
Sol: (a) Polytene.
3. Lampbrush chromosomes occur in___________.
(a) Oocytes
(b) Cancer cells
(c) Lymph glands
(d) Salivary glands
Sol: (a) Oocytes.
4. Which of the following is true about the Chromatids?
(a) It is a haploid chromosome
(b) It is a complete chromosome
(c) It is a duplicate chromosome
(d) It is one-half of the replicated chromosome
Sol: (d) It is one-half of the replicated chromosome.
5. The centromere is that part of the chromosome where___________.
(a) Nicking occurs
(b) Chromatids are attached
(c) Nucleoli are formed
(d) Crossing-over takes place
Sol: (b) Chromatids are attached.
6. The ends of the chromosome are called ___________.
(a) Satellites
(b) Centromeres
(c) Telomeres
(d) Kinetochore
Sol: (c) Telomeres.
7. Chromosomes were first observed by___________.
(a) Fleming
(b) Waldeyer
(c) Strasburger
Sol: (d) Hoffmeister.
8. A chromosome with sub-terminal centromere is___________.
(a) Acrocentric
(b) Acentric
(c) Metacentric
(d) Telocentric
Sol: (a) Acrocentric.
9. The giant chromosome with a number of chromonemeta is___________.
(a) Hetrochromosome
(b) Polytene chromosome
(c) Lampbrush chromosome
(d) Supernumerary chromosome
Sol: (b) Polytene chromosome.
10. A chromosome with centromere near the middle is called___________.
(a) Metacentric
(b) Acrocentric
(c) Telocentric
(d) Submetacentric
Sol: (d) Submetacentric.
11. Puffs or balbiani rings in the salivary gland chromosome are the sites of___________.
(a) Protein synthesis
(b) RNA synthesis
(c) DNA replication
(d) DNA duplication
Sol: (b) RNA synthesis.
12. Chromosomal theory of inheritance was proposed by___________.
(a) Sutton in 1902
(b) Boveri in 1902
(c) Correns in 1909
(d) Sutton and Boveri in 1902
Sol: (d) Sutton and Boveri in 1902.
13. More than 200 chromosomes occur in___________.
(c) Chicken
(d) Gorilla
Sol: (b) Amoeba.
14. A colour blind daughter may be born if the___________.
(a) Father is normal and the mother is a carrier
(b) Father is normal and the mother is colour blind
(c) Father is colour blind and mother is a carrier
(d) Father is colour blind and mother is normal
Sol: (c) Father is colour blind and mother is a carrier.
15. A somatic cell in a human male contains___________.
(a) No gene on the sex chromosome
(b) Genes on only on sex chromosomes
(c) Two genes for every sex-linked character
(d) Only one sex-linked gene for each character
Sol: (d) Only one sex-linked gene for each character.
16. The blue-green algae and bacteria contain ___________.
(a) Three linkage groups
(b) Two linkage groups
(c) One linkage group
(d) None of the above
Sol: (c) One linkage group.
17. What is the number of linkage groups in the Drosophila?
Sol: (b) Four.
18. Gene for colour blindness in man is located on ___________.
(a) Both X and Y chromosome
(b) Y-chromosome only
(c) X-chromosome only
(d) Either X-chromosome or Y-chromosome
Sol: (c) X-chromosome only.
19. Chromosomal constitution in human females can be best written as___________.
Sol: (d) 44A+XX.
20. Which of the following disease is sex-linked?
(a) Hepatitis
(b) Leukaemia
(c) Malignancy
(d) Colour blindness
Sol: (d) Colour blindness.
Stay tuned with BYJU’S Biology for more MCQs and other Biology related topics.
BIOLOGY Related Links | |
Leave a Comment Cancel reply
Your Mobile number and Email id will not be published. Required fields are marked *
Request OTP on Voice Call
Post My Comment

Register with BYJU'S & Download Free PDFs
Register with byju's & watch live videos.

COMMENTS
Modes of Extrachromosomal Inheritance. 1. Uniparental inheritance. Uniparental inheritance refers to a mode of inheritance where genetic material or traits are inherited from a single parent, either the mother or the father. The genomes of extrachromosomal organelles are maternally inherited in most eukaryotes.
The extrachromosomal DNA is inherited from the maternal side because the female gamete contains more cytoplasm than the male gamete. 4. All the progenies obtained by this inheritance have the phenotype of only one parent, i.e., the mother. 5. The extranuclear genes present in the mitochondria and plastids cannot be mapped to the chromosomes in ...
Location. Found in organelles like mitochondria and chloroplasts. Found in the nucleus within chromosomes. Mode of Transmission. Typically maternal (e.g., mitochondrial DNA) Genetic traits on nuclear chromosomes generally follow Mendelian patterns of inheritance. Examples. Mitochondrial DNA, chloroplast DNA. Nuclear DNA in chromosomes.
Chromosomal inheritance is the process by which genetic information is passed down from parent to offspring through the transmission of chromosomes. These chromosomes contain the genes that determine various traits and characteristics in organisms. The study of chromosomal inheritance has helped scientists uncover the complex mechanisms by which genes are inherited and expressed.
Maternal influence. Also known as predetermination or delayed inheritance; refers to the effect of the maternal parent's genotype on the phenotype of her offspring. Sex-linked inheritance. The phenotypic expression of an allele related to the sex chromosome of the individual. This type of inheritance is different from the inheritance of ...
The X chromosome contains 867 identified genes; most of these genes are responsible for the development of tissues like bone, neural, blood, hepatic, renal, retina, ears, ear, cardiac, skin, and teeth. There are at least 533 disorders due to the involvement of the genes on the X chromosome.[1] A 'trait' or 'disorder' determined by a gene on the X chromosome demonstrates X-linked inheritance.
Abstract. As early as 1909 Carl Correns, one of the rediscoverers of the Mendelian laws, recognized that the nucleus did not have a monopoly on heredity. He was able to show that the cytoplasm also carries hereditary determinants. In the years following, many cases of extrachromosomal inheritance were described in plants and animals.
Abstract. Oncogene amplification on extrachromosomal DNA (ecDNA) is a common event, driving aggressive tumor growth, drug resistance and shorter survival. Currently, the impact of nonchromosomal ...
1.1.2. Telomeric circles (t-circles) In 1995, Nosek et al. reported the discovery of inverted terminal repeats that were made up of tandemly repeating units in yeast type 2 linear mitochondrial genomes (mtDNA).Taking Candida parapsilosis as an example, the terminus is comprised of a 738 bp repeating unit, with a 5′ single-stranded extension of about 110 nucleotides that is accessible to the ...
While the term genome includes all of the chromosomal hereditary determinants, the extrachromosomal hereditary ... The expressions" cytoplasmic" and" extra nuclear inheritance" as well as "extra chromosomal inheritance" have all been used in the literature. Vve prefer "extrachromosomal inheritance" in order to emphasize that the 439 K. Esser ...
In 1902 and 1903, Sutton and Boveri published independent papers proposing what we now call the chromosome theory of inheritance. This theory states that individual genes are found at specific locations on particular chromosomes, and that the behavior of chromosomes during meiosis can explain why genes are inherited according to Mendel's laws ...
7. Term Paper on the Inheritance of Sex: Whether a child is born male or female is determined at the moment of fertilisation. Of the 23 pairs of chromosomes in a human nucleus, one pair is known as the sex chromosomes. In the female, the sex chromosomes are identical and are called 'X' chromosomes. In the male, they are not identical.
BIOLOGY I. Chapter 15 - The Chromosomal Basis of Inheritance 3 The chromosomal basis of Mendel's laws. Correlation of the results of one of Mendel's dihybrid crosses with the behavior of chromosomes during meiosis. Law of segregation: The two alleles for each gene separate during gamete formation. As an example, follow the fate of the
The newly discovered chromosomal independence during meiosis meant that the number of possible chromosomal combinations for each gamete could be calculated based on the number of chromosomes in ...
Human genes are arranged on 23 pairs of chromosomes, but in cancer, tumor-promoting genes and regulatory elements can free themselves from chromosomes and relocate to circular, extrachromosomal pieces of DNA (ecDNA). ecDNA, because of its nonchromosomal inheritance, drives high-copy-number oncogene amplification and enables tumors to evolve their genomes rapidly.
The correct chromosome number in humans was determined and confirmed in 1956 [1], [2]. The behavior of chromosomes during meiotic cell division provides the basis for the Mendelian laws of inheritance, whereas their abnormal behavior in cell division leads to abnormalities of chromosome number. In this chapter, we examine the current ...
The Chromosomal Theory of Inheritance. The chromosomal theory of inheritance was given by Boveri and Sutton in the early 1900s. It is the fundamental theory of genetics. According to this theory, genes are the units of heredity and are found in the chromosomes. Chromosomal Theory of Inheritance came into existence long after Mendelian genetics.
What of the following provides the best evidence that DNA is the genetic material? Choose 1 answer: Choose 1 answer: (Choice A) Biomolecular composition of chromosomes. A. Biomolecular composition of chromosomes. (Choice B) Transformation using heat-inactivated bacteria.
Important Questions for Class 12 Chapter 5 Principles of Inheritance and Variations. Inheritance is the transfer of genes from parents to the offsprings. The principles of inheritance and variation were explained by Gregor Mendel in his experiments on a pea plant. He stated three laws of inheritance on the basis of his observations with the pea ...
Test your knowledge with important MCQs on the chromosomal Basis Of Inheritance. These MCQs are beneficial for competitive exams too. Explore more MCQs at BYJU'S. ... CBSE Sample Papers. CBSE Sample Papers for Class 6; CBSE Sample Papers for Class 7; ... The term chromosome was coined by _____. (a) Sutton (b) Boveri