The origin of life on Earth, explained
The origin of life on Earth stands as one of the great mysteries of science. Various answers have been proposed, all of which remain unverified. To find out if we are alone in the galaxy, we will need to better understand what geochemical conditions nurtured the first life forms. What water, chemistry and temperature cycles fostered the chemical reactions that allowed life to emerge on our planet? Because life arose in the largely unknown surface conditions of Earth’s early history, answering these and other questions remains a challenge.
Several seminal experiments in this topic have been conducted at the University of Chicago, including the Miller-Urey experiment that suggested how the building blocks of life could form in a primordial soup.

Jump to a section:
- When did life on Earth begin?
Where did life on Earth begin?
What are the ingredients of life on earth, what are the major scientific theories for how life emerged, what is chirality and why is it biologically important, what research are uchicago scientists currently conducting on the origins of life, when did life on earth begin .
Earth is about 4.5 billion years old. Scientists think that by 4.3 billion years ago, Earth may have developed conditions suitable to support life. The oldest known fossils, however, are only 3.7 billion years old. During that 600 million-year window, life may have emerged repeatedly, only to be snuffed out by catastrophic collisions with asteroids and comets.
The details of those early events are not well preserved in Earth’s oldest rocks. Some hints come from the oldest zircons, highly durable minerals that formed in magma. Scientists have found traces of a form of carbon—an important element in living organisms— in one such 4.1 billion-year-old zircon . However, it does not provide enough evidence to prove life’s existence at that early date.
Two possibilities are in volcanically active hydrothermal environments on land and at sea.
Some microorganisms thrive in the scalding, highly acidic hot springs environments like those found today in Iceland, Norway and Yellowstone National Park. The same goes for deep-sea hydrothermal vents. These chimney-like vents form where seawater comes into contact with magma on the ocean floor, resulting in streams of superheated plumes. The microorganisms that live near such plumes have led some scientists to suggest them as the birthplaces of Earth’s first life forms.
Organic molecules may also have formed in certain types of clay minerals that could have offered favorable conditions for protection and preservation. This could have happened on Earth during its early history, or on comets and asteroids that later brought them to Earth in collisions. This would suggest that the same process could have seeded life on planets elsewhere in the universe.
The recipe consists of a steady energy source, organic compounds and water.
Sunlight provides the energy source at the surface, which drives photosynthesis. On the ocean floor, geothermal energy supplies the chemical nutrients that organisms need to live.
Also crucial are the elements important to life . For us, these are carbon, hydrogen, oxygen, nitrogen, and phosphorus. But there are several scientific mysteries about how these elements wound up together on Earth. For example, scientists would not expect a planet that formed so close to the sun to naturally incorporate carbon and nitrogen. These elements become solid only under very cold temperatures, such as exist in the outer solar system, not nearer to the sun where Earth is. Also, carbon, like gold, is rare at the Earth’s surface. That’s because carbon chemically bonds more often with iron than rock. Gold also bonds more often with metal, so most of it ends up in the Earth’s core. So, how did the small amounts found at the surface get there? Could a similar process also have unfolded on other planets?
The last ingredient is water. Water now covers about 70% of Earth’s surface, but how much sat on the surface 4 billion years ago? Like carbon and nitrogen, water is much more likely to become a part of solid objects that formed at a greater distance from the sun. To explain its presence on Earth, one theory proposes that a class of meteorites called carbonaceous chondrites formed far enough from the sun to have served as a water-delivery system.
There are several theories for how life came to be on Earth. These include:
Life emerged from a primordial soup
As a University of Chicago graduate student in 1952, Stanley Miller performed a famous experiment with Harold Urey, a Nobel laureate in chemistry. Their results explored the idea that life formed in a primordial soup.
Miller and Urey injected ammonia, methane and water vapor into an enclosed glass container to simulate what were then believed to be the conditions of Earth’s early atmosphere. Then they passed electrical sparks through the container to simulate lightning. Amino acids, the building blocks of proteins, soon formed. Miller and Urey realized that this process could have paved the way for the molecules needed to produce life.
Scientists now believe that Earth’s early atmosphere had a different chemical makeup from Miller and Urey’s recipe. Even so, the experiment gave rise to a new scientific field called prebiotic or abiotic chemistry, the chemistry that preceded the origin of life. This is the opposite of biogenesis, the idea that only a living organism can beget another living organism.
Seeded by comets or meteors
Some scientists think that some of the molecules important to life may be produced outside the Earth. Instead, they suggest that these ingredients came from meteorites or comets.
“A colleague once told me, ‘It’s a lot easier to build a house out of Legos when they’re falling from the sky,’” said Fred Ciesla, a geophysical sciences professor at UChicago. Ciesla and that colleague, Scott Sandford of the NASA Ames Research Center, published research showing that complex organic compounds were readily produced under conditions that likely prevailed in the early solar system when many meteorites formed.
Meteorites then might have served as the cosmic Mayflowers that transported molecular seeds to Earth. In 1969, the Murchison meteorite that fell in Australia contained dozens of different amino acids—the building blocks of life.
Comets may also have offered a ride to Earth-bound hitchhiking molecules, according to experimental results published in 2001 by a team of researchers from Argonne National Laboratory, the University of California Berkeley, and Lawrence Berkeley National Laboratory. By showing that amino acids could survive a fiery comet collision with Earth, the team bolstered the idea that life’s raw materials came from space.
In 2019, a team of researchers in France and Italy reported finding extraterrestrial organic material preserved in the 3.3 billion-year-old sediments of Barberton, South Africa. The team suggested micrometeorites as the material’s likely source. Further such evidence came in 2022 from samples of asteroid Ryugu returned to Earth by Japan’s Hayabusa2 mission. The count of amino acids found in the Ryugu samples now exceeds 20 different types .
In 1953, UChicago researchers published a landmark paper in the Journal of Biological Chemistry that marked the discovery of the pro-chirality concept , which pervades modern chemistry and biology. The paper described an experiment showing that the chirality of molecules—or “handedness,” much the way the right and left hands differ from one another—drives all life processes. Without chirality, large biological molecules such as proteins would be unable to form structures that could be reproduced.
Today, research on the origin of life at UChicago is expanding. As scientists have been able to find more and more exoplanets—that is, planets around stars elsewhere in the galaxy—the question of what the essential ingredients for life are and how to look for signs of them has heated up.
Nobel laureate Jack Szostak joined the UChicago faculty as University Professor in Chemistry in 2022 and will lead the University’s new interdisciplinary Origins of Life Initiative to coordinate research efforts into the origin of life on Earth. Scientists from several departments of the Physical Sciences Division are joining the initiative, including specialists in chemistry, astronomy, geology and geophysics.
“Right now we are getting truly unprecedented amounts of data coming in: Missions like Hayabusa and OSIRIS-REx are bringing us pieces of asteroids, which helps us understand the conditions that form planets, and NASA’s new JWST telescope is taking astounding data on the solar system and the planets around us ,” said Prof. Ciesla. “I think we’re going to make huge progress on this question.”
Last updated Sept. 19, 2022.
Faculty Experts
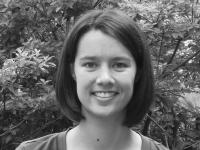
Clara Blättler
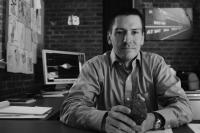
Fred Ciesla
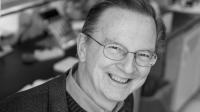
Jack Szostak
Recommended Stories
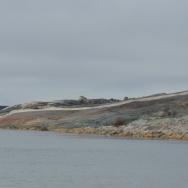
Earth could have supported crust, life earlier than thought
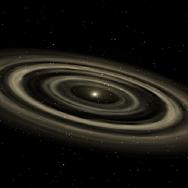
Earth’s building blocks formed during the solar system’s first…
Additional Resources
The Origins of Life Speaker Series
Recommended Podcasts
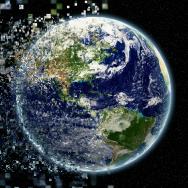
Big Brains podcast: Unraveling the mystery of life’s origins on Earth
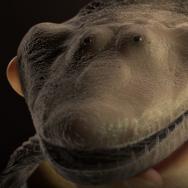
Discovering the Missing Link with Neil Shubin (Ep. 1)
More Explainers
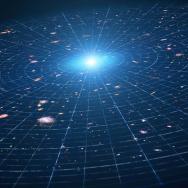
Dark energy, explained
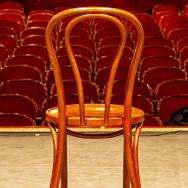
Improv, Explained
Related Topics
Latest news, at 2024 chicago quantum summit, leaders temper hype, hail momentum of global quantum effort.
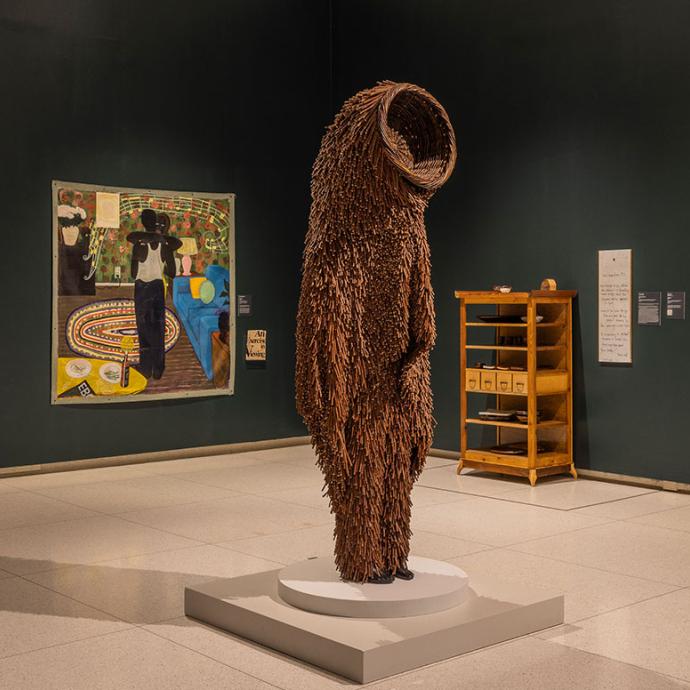
Smart Museum celebrates 50 years of examining the world through art

Botany Pond
Botany Pond restoration revitalizes beloved campus landmark

Astronomy and Astrophysics
The long and strange lives of Enrico Fermi’s accelerator building at the University of Chicago
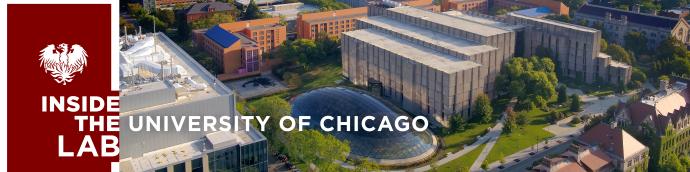
Go 'Inside the Lab' at UChicago
Explore labs through videos and Q&As with UChicago faculty, staff and students

Humanities Day
Prof. Philip V. Bohlman to address ‘doing good’ through music during Humanities Day keynote
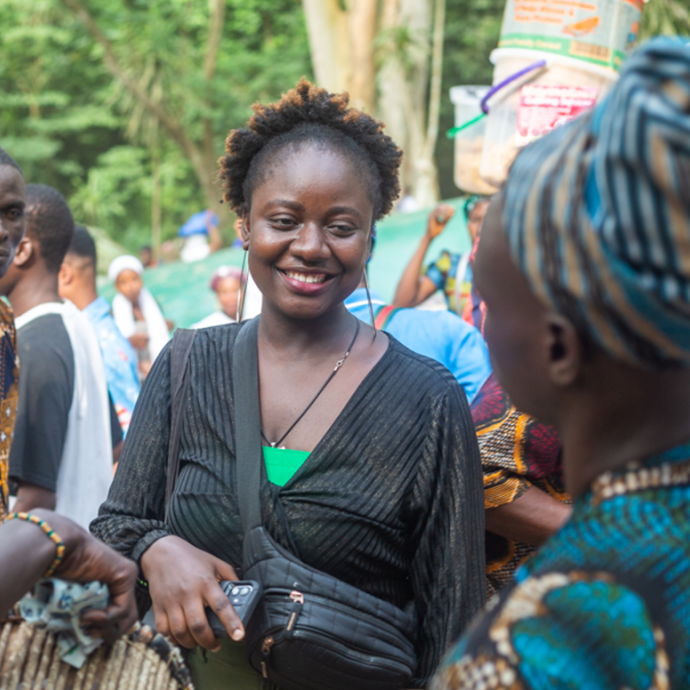
Dispatches from Abroad
From Andean forests to ancient sites in Turkey, UChicagoans spent the summer abroad
Around uchicago.

Nobel Prize
UChicago alum John Jumper shares Nobel Prize for model to predict protein structures
Two uchicago scientists honored with 2024 department of energy early career awa….
New Program
UChicago offers new master’s program in environmental science
Artificial Intelligence
NSF, Simons Foundation launch $20 million National AI Research Institute in Ast…
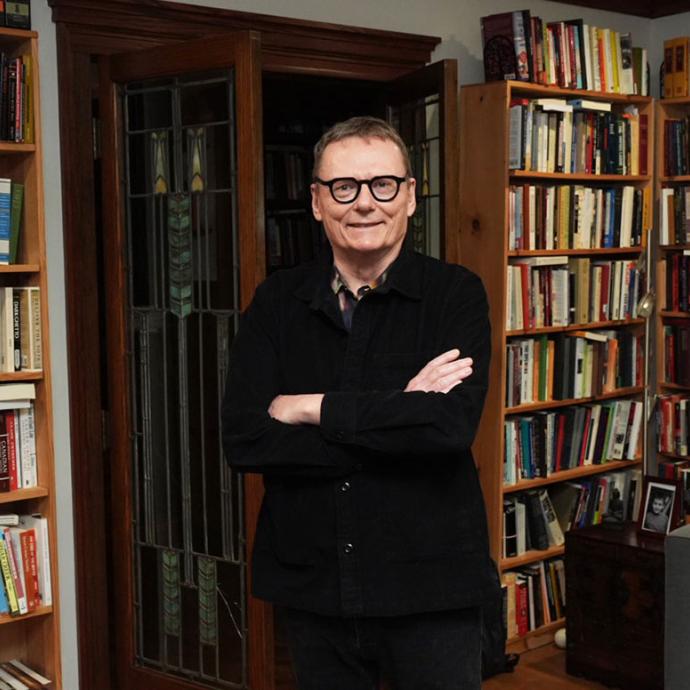
James A. Robinson shares 2024 Nobel Prize for research on global inequality

Big Brains podcast
Big Brains podcast: 2024 Nobel laureate explains what makes countries fail or succeed, with James A. Robinson
Film History
“Throughout my time at UChicago, I’ve sought to provide opportunities to share scholarship with the public”
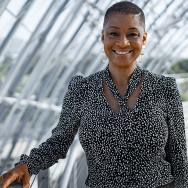
2024 South Side Science Festival offers up fun and inspiration
September 1, 2009
13 min read
The Origin of Life on Earth
Fresh clues hint at how the first living organisms arose from inanimate matter
By Alonso Ricardo & Jack W. Szostak
Every living cell, even the simplest bacterium, teems with molecular contraptions that would be the envy of any nanotechnologist. As they incessantly shake or spin or crawl around the cell, these machines cut, paste and copy genetic molecules, shuttle nutrients around or turn them into energy, build and repair cellular membranes, relay mechanical, chemical or electrical messages—the list goes on and on, and new discoveries add to it all the time.
It is virtually impossible to imagine how a cell’s machines, which are mostly protein-based catalysts called enzymes, could have formed spontaneously as life first arose from nonliving matter around 3.7 billion years ago. To be sure, under the right conditions some building blocks of proteins, the amino acids, form easily from simpler chemicals, as Stanley L. Miller and Harold C. Urey of the University of Chicago discovered in pioneering experiments in the 1950s. But going from there to proteins and enzymes is a different matter.
A cell’s protein-making process involves complex enzymes pulling apart the strands of DNA’s double helix to extract the information contained in genes (the blueprints for the proteins) and translate it into the finished product. Thus, explaining how life began entails a serious paradox: it seems that it takes proteins—as well as the information now stored in DNA—to make proteins.
On supporting science journalism
If you're enjoying this article, consider supporting our award-winning journalism by subscribing . By purchasing a subscription you are helping to ensure the future of impactful stories about the discoveries and ideas shaping our world today.
On the other hand, the paradox would disappear if the first organisms did not require proteins at all. Recent experiments suggest it would have been possible for genetic molecules similar to DNA or to its close relative RNA to form spontaneously. And because these molecules can curl up in different shapes and act as rudimentary catalysts, they may have become able to copy themselves—to reproduce—without the need for proteins. The earliest forms of life could have been simple membranes made of fatty acids—also structures known to form spontaneously—that enveloped water and these self-replicating genetic molecules. The genetic material would encode the traits that each generation handed down to the next, just as DNA does in all things that are alive today. Fortuitous mutations, appearing at random in the copying process, would then propel evolution, enabling these early cells to adapt to their environment, to compete with one another, and eventually to turn into the life-forms we know.
The actual nature of the first organisms and the exact circumstances of the origin of life may be forever lost to science. But research can at least help us understand what is possible. The ultimate challenge is to construct an artificial organism that can reproduce and evolve. Creating life anew will certainly help us understand how life can start, how likely it is that it exists on other worlds and, ultimately, what life is.
Got to Start Somewhere One of the most difficult and interesting mysteries surrounding the origin of life is exactly how the genetic material could have formed starting from simpler molecules present on the early earth. Judging from the roles that RNA has in modern cells, it seems likely that RNA appeared before DNA. When modern cells make proteins, they first copy genes from DNA into RNA and then use the RNA as a blueprint to make proteins. This last stage could have existed independently at first. Later on, DNA could have appeared as a more permanent form of storage, thanks to its superior chemical stability.
Investigators have one more reason for thinking that RNA came before DNA. The RNA versions of enzymes, called ribozymes, also serve a pivotal role in modern cells. The structures that translate RNA into proteins are hybrid RNA-protein machines, and it is the RNA in them that does the catalytic work. Thus, each of our cells appears to carry in its ribosomes “fossil” evidence of a primordial RNA world.
Much research, therefore, has focused on understanding the possible origin of RNA. Genetic molecules such as DNA and RNA are polymers (strings of smaller molecules) made of building blocks called nucleotides. In turn, nucleotides have three distinct components: a sugar, a phosphate and a nucleobase. Nucleobases come in four types and constitute the alphabet in which the polymer encodes information. In a DNA nucleotide the nucleobase can be A, G, C or T, standing for the molecules adenine, guanine, cytosine or thymine; in the RNA alphabet the letter U, for uracil, replaces the T. The nucleobases are nitrogen-rich compounds that bind to one another according to a simple rule; thus, A pairs with U (or T), and G pairs with C. Such base pairs form the rungs of DNA’s twisted ladder—the familiar double helix—and their exclusive pairings are crucial for faithfully copying the information so a cell can reproduce. Meanwhile the phosphate and sugar molecules form the backbone of each strand of DNA or RNA.
Nucleobases can assemble spontaneously, in a series of steps, from cyanide, acetylene and water—simple molecules that were certainly present in the primordial mix of chemicals. Sugars are also easy to assemble from simple starting materials. It has been known for well over 100 years that mixtures of many types of sugar molecules can be obtained by warming an alkaline solution of formaldehyde, which also would have been available on the young planet. The problem, however, is how to obtain the “right” kind of sugar—ribose, in the case of RNA—to make nucleotides. Ribose, along with three closely related sugars, can form from the reaction of two simpler sugars that contain two and three carbon atoms, respectively. Ribose’s ability to form in that way does not solve the problem of how it became abundant on the early earth, however, because it turns out that ribose is unstable and rapidly breaks down in an even mildly alkaline solution. In the past, this observation has led many researchers to conclude that the first genetic molecules could not have contained ribose. But one of us (Ricardo) and others have discovered ways in which ribose could have been stabilized.
The phosphate part of nucleotides presents another intriguing puzzle. Phosphorus—the central element of the phosphate group—is abundant in the earth’s crust but mostly in minerals that do not dissolve readily in water, where life presumably originated. So it is not obvious how phosphates would have gotten into the prebiotic mix. The high temperatures of volcanic vents can convert phosphate-containing minerals to soluble forms of phosphate, but the amounts released, at least near modern volcanoes, are small. A completely different potential source of phosphorus compounds is schreibersite, a mineral commonly found in certain meteors.
In 2005 Matthew Pasek and Dante Lauretta of the University of Arizona discovered that the corrosion of schreibersite in water releases its phosphorus component. This pathway seems promising because it releases phosphorus in a form that is both much more soluble in water than phosphate and much more reactive with organic (carbon-based) compounds.
Some Assembly Required Given that we have at least an outline of potential pathways leading to the nucleobases, sugars and phosphate, the next logical step would be to properly connect these components. This step, however, is the one that has caused the most intense frustration in prebiotic chemistry research for the past several decades. Simply mixing the three components in water does not lead to the spontaneous formation of a nucleotide—largely because each joining reaction also involves the release of a water molecule, which does not often occur spontaneously in a watery solution. For the needed chemical bonds to form, energy must be supplied, for example, by adding energy-rich compounds that aid in the reaction. Many such compounds may have existed on the early earth. In the laboratory, however, reactions powered by such molecules have proved to be inefficient at best and in most cases completely unsuccessful.
This spring—to the field’s great excitement—John Sutherland and his co-workers at the University of Manchester in England announced that they found a much more plausible way that nucleotides could have formed, which also sidesteps the issue of ribose’s instability. These creative chemists abandoned the tradition of attempting to make nucleotides by joining a nucleobase, sugar and phosphate. Their approach relies on the same simple starting materials employed previously, such as derivatives of cyanide, acetylene and formaldehyde. But instead of forming nucleobase and ribose separately and then trying to join them, the team mixed the starting ingredients together, along with phosphate. A complex web of reactions—with phosphate acting as a crucial catalyst at several steps along the way—produced a small molecule called 2-aminooxazole, which can be viewed as a fragment of a sugar joined to a piece of a nucleobase.
A crucial feature of this small, stable molecule is that it is very volatile. Perhaps small amounts of 2-aminooxazole formed together with a mixture of other chemicals in a pond on the early earth; once the water evaporated, the 2-aminooxazole vaporized, only to condense elsewhere, in a purified form. There it would accumulate as a reservoir of material, ready for further chemical reactions that would form a full sugar and nucleobase attached to each other.
Another important and satisfying aspect of this chain of reactions is that some of the early-stage by-products facilitate transformations at later stages in of the process. Elegant as it is, the pathway does not generate exclusively the “correct” nucleotides: in some cases, the sugar and nucleobase are not joined in the proper spatial arrangement. But amazingly, exposure to ultraviolet light—intense solar UV rays hit shallow waters on the early earth—destroys the “incorrect” nucleotides and leaves behind the “correct” ones. The end result is a remarkably clean route to the C and U nucleotides. Of course, we still need a route to G and A, so challenges remain. But the work by Sutherland’s team is a major step toward explaining how a molecule as complex as RNA could have formed on the early earth.
Some Warm, Little Vial Once we have nucleotides, the final step in the formation of an RNA molecule is polymerization: the sugar of one nucleotide forms a chemical bond with the phosphate of the next, so that nucleotides string themselves together into a chain. Once again, in water the bonds do not form spontaneously and instead require some external energy. By adding various chemicals to a solution of chemically reactive versions of the nucleotides, researchers have been able to produce short chains of RNA, two to 40 nucleotides long. In the late 1990s Jim Ferris and his co-workers at the Rensselaer Polytechnic Institute showed that clay minerals enhance the process, producing chains of up to 50 or so nucleotides. (A typical gene today is thousands to millions of nucleotides long.) The minerals’ intrinsic ability to bind nucleotides brings reactive molecules close together, thereby facilitating the formation of bonds between them.
The discovery reinforced the suggestion by some researchers that life may have started on mineral surfaces, perhaps in clay-rich muds at the bottom of pools of water formed by hot springs [see “Life's Rocky Start,” by Robert M. Hazen; Scientific American, April 2001].
Certainly finding out how genetic polymers first arose would not by itself solve the problem of the origin of life. To be “alive,” organisms must be able to go forth and multiply—a process that includes copying genetic information. In modern cells enzymes, which are protein-based, carry out this copying function.
But genetic polymers, if they are made of the right sequences of nucleotides, can fold into complex shapes and can catalyze chemical reactions, just as today’s enzymes do. Hence, it seems plausible that RNA in the very first organisms could have directed its own replication. This notion has inspired several experiments, both at our lab and at David Bartel’s lab at the Massachusetts Institute of Technology, in which we “evolved” new ribozymes.
We started with trillions of random RNA sequences. Then we selected the ones that had catalytic properties, and we made copies of those. At each round of copying some of the new RNA strands underwent mutations that turned them into more efficient catalysts, and once again we singled those out for the next round of copying. By this directed evolution we were able to produce ribozymes that can catalyze the copying of relatively short strands of other RNAs, although they fall far short of being able to copy polymers with their own sequences into progeny RNAs.
Recently the principle of RNA self-replication received a boost from Tracey Lincoln and Gerald Joyce of the Scripps Research Institute, who evolved two RNA ribozymes, each of which could make copies of the other by joining together two shorter RNA strands. Unfortunately, success in the experiments required the presence of preexisting RNA pieces that were far too long and complex to have accumulated spontaneously. Still, the results suggest that RNA has the raw catalytic power to catalyze its own replication.
Is there a simpler alternative? We and others are now exploring chemical ways of copying genetic molecules without the aid of catalysts. In recent experiments, we started with single, “template” strands of DNA. (We used DNA because it is cheaper and easier to work with, but we could just as well have used RNA.) We mixed the templates in a solution containing isolated nucleotides to see if nucleotides would bind to the template through complementary base pairing (A joining to T and C to G) and then polymerize, thus forming a full double strand. This would be the first step to full replication: once a double strand had formed, separation of the strands would allow the complement to serve as a template for copying the original strand. With standard DNA or RNA, the process is exceedingly slow. But small changes to the chemical structure of the sugar component—changing one oxygen-hydrogen pair to an amino group (made of nitrogen and hydrogen)—made the polymerization hundreds of times faster, so that complementary strands formed in hours instead of weeks. The new polymer behaved much like classic RNA despite having nitrogen-phosphorus bonds instead of the normal oxygen-phosphorus bonds.
Boundary Issues If we assume for the moment that the gaps in our understanding of the chemistry of life’s origin will someday be filled, we can begin to consider how molecules might have interacted to assemble into the first cell-like structures, or “protocells.”
The membranes that envelop all modern cells consist primarily of a lipid bilayer: a double sheet of such oily molecules as phospholipids and cholesterol. Membranes keep a cell’s components physically together and form a barrier to the uncontrolled passage of large molecules. Sophisticated proteins embedded in the membrane act as gatekeepers and pump molecules in and out of the cell, while other proteins assist in the construction and repair of the membrane. How on earth could a rudimentary protocell, lacking protein machinery, carry out these tasks?
Primitive membranes were probably made of simpler molecules, such as fatty acids (which are one component of the more complex phospholipids). Studies in the late 1970s showed that membranes could indeed assemble spontaneously from plain fatty acids, but the general feeling was that these membranes would still pose a formidable barrier to the entry of nucleotides and other complex nutrients into the cell. This notion suggested that cellular metabolism had to develop first, so that cells could synthesize nucleotides for themselves. Work in our lab has shown, however, that molecules as large as nucleotides can in fact easily slip across membranes as long as both nucleotides and membranes are simpler, more “primitive” versions of their modern counterparts.
This finding allowed us to carry out a simple experiment modeling the ability of a protocell to copy its genetic information using environmentally supplied nutrients. We prepared fatty acid–based membrane vesicles containing a short piece of single-stranded DNA. As before, the DNA was meant to serve as a template for a new strand. Next, we exposed these vesicles to chemically reactive versions of nucleotides. The nucleotides crossed the membrane spontaneously and, once inside the model protocell, lined up on the DNA strand and reacted with one another to generate a complementary strand. The experiment supports the idea that the first protocells contained RNA (or something similar to it) and little else and replicated their genetic material without enzymes.
Let There Be Division For protocells to start reproducing, they would have had to be able to grow, duplicate their genetic contents and divide into equivalent “daughter” cells. Experiments have shown that primitive vesicles can grow in at least two distinct ways. In pioneering work in the 1990s, Pier Luigi Luisi and his colleagues at the Swiss Federal Institute of Technology in Zurich added fresh fatty acids to the water surrounding such vesicles. In response, the membranes incorporated the fatty acids and grew in surface area. As water and dissolved substances slowly entered the interior, the cell’s volume also increased.
A second approach, which was explored in our lab by then graduate student Irene Chen, involved competition between protocells. Model protocells filled with RNA or similar materials became swollen, an osmotic effect resulting from the attempt of water to enter the cell and equalize its concentration inside and outside. The membrane of such swollen vesicles thus came under tension, and this tension drove growth, because adding new molecules relaxes the tension on the membrane, lowering the energy of the system. In fact, swollen vesicles grew by stealing fatty acids from relaxed neighboring vesicles, which shrank.
In the past year Ting Zhu, a graduate student in our lab, has observed the growth of model protocells after feeding them fresh fatty acids. To our amazement, the initially spherical vesicles did not grow simply by getting larger. Instead they first extended a thin filament. Over about half an hour, this protruding filament grew longer and thicker, gradually transforming the entire initial vesicle into a long, thin tube. This structure was quite delicate, and gentle shaking (such as might occur as wind generates waves on a pond) caused it to break into a number of smaller, spherical daughter protocells, which then grew larger and repeated the cycle.
Given the right building blocks, then, the formation of protocells does not seem that difficult: membranes self-assemble, genetic polymers self-assemble, and the two components can be brought together in a variety of ways, for example, if the membranes form around preexisting polymers. These sacs of water and RNA will also grow, absorb new molecules, compete for nutrients, and divide. But to become alive, they would also need to reproduce and evolve. In particular, they need to separate their RNA double strands so each single strand can act as a template for a new double strand that can be handed down to a daughter cell.
This process would not have started on its own, but it could have with a little help. Imagine, for example, a volcanic region on the otherwise cold surface of the early earth (at the time, the sun shone at only 70 percent of its current power). There could be pools of cold water, perhaps partly covered by ice but kept liquid by hot rocks. The temperature differences would cause convection currents, so that every now and then protocells in the water would be exposed to a burst of heat as they passed near the hot rocks, but they would almost instantly cool down again as the heated water mixed with the bulk of the cold water. The sudden heating would cause a double helix to separate into single strands. Once back in the cool region, new double strands—copies of the original one—could form as the single strands acted as templates.
As soon as the environment nudged protocells to start reproducing, evolution kicked in. In particular, at some point some of the RNA sequences mutated, becoming ribozymes that sped up the copying of RNA—thus adding a competitive advantage. Eventually ribozymes began to copy RNA without external help.
It is relatively easy to imagine how RNA-based protocells may have then evolved [see box above]. Metabolism could have arisen gradually, as new ribozymes enabled cells to synthesize nutrients internally from simpler and more abundant starting materials. Next, the organisms might have added protein making to their bag of chemical tricks.
With their astonishing versatility, proteins would have then taken over RNA’s role in assisting genetic copying and metabolism. Later, the organisms would have “learned” to make DNA, gaining the advantage of possessing a more robust carrier of genetic information. At that point, the RNA world became the DNA world, and life as we know it began.
Note: This article was originally printed with the title, "Origin of Life on Earth."
Thank you for visiting nature.com. You are using a browser version with limited support for CSS. To obtain the best experience, we recommend you use a more up to date browser (or turn off compatibility mode in Internet Explorer). In the meantime, to ensure continued support, we are displaying the site without styles and JavaScript.
- View all journals
- Explore content
- About the journal
- Publish with us
- Sign up for alerts
- INNOVATIONS IN
- 09 May 2018

How Did Life Begin?
- Jack Szostak 0
Jack Szostak is a professor of genetics at Harvard Medical School and one of the recipients of the 2009 Nobel Prize in Physiology or Medicine.
You can also search for this author in PubMed Google Scholar
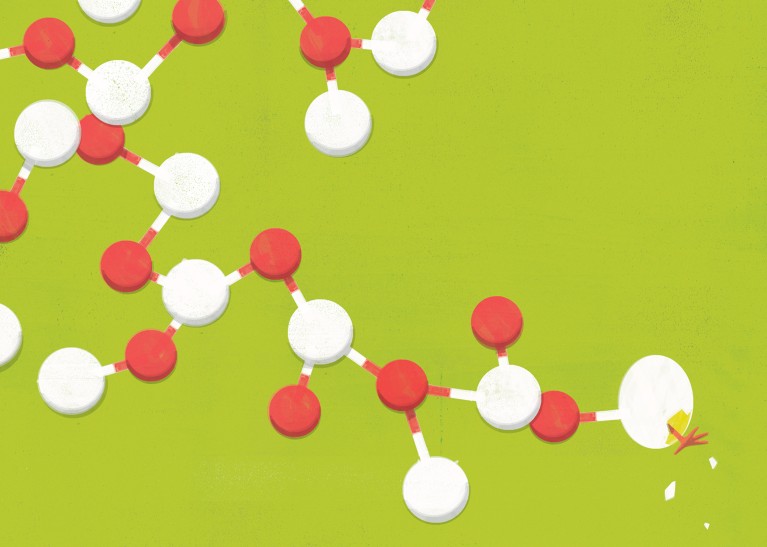
Illustration by Chris Gash
Is the existence of life on Earth a lucky fluke or an inevitable consequence of the laws of nature? Is it simple for life to emerge on a newly formed planet, or is it the virtually impossible product of a long series of unlikely events? Advances in fields as disparate as astronomy, planetary science and chemistry now hold promise that answers to such profound questions may be around the corner. If life turns out to have emerged multiple times in our galaxy, as scientists are hoping to discover, the path to it cannot be so hard. Moreover, if the route from chemistry to biology proves simple to traverse, the universe could be teeming with life.
The discovery of thousands of exoplanets has sparked a renaissance in origin-of-life studies. In a stunning surprise, almost all the newly discovered solar systems look very different from our own. Does that mean something about our own, very odd, system favors the emergence of life? Detecting signs of life on a planet orbiting a distant star is not going to be easy, but the technology for teasing out subtle “biosignatures” is developing so rapidly that with luck we may see distant life within one or two decades.
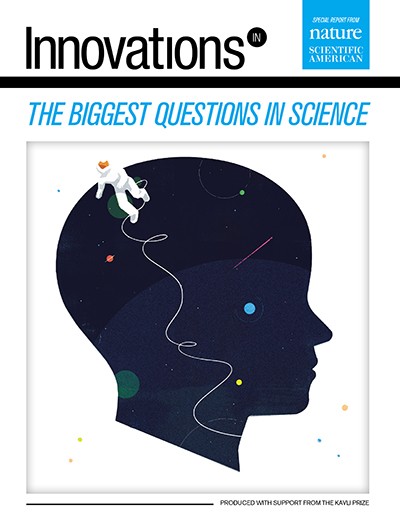
Innovations In The Biggest Questions In Science
To understand how life might begin, we first have to figure out how—and with what ingredients—planets form. A new generation of radio telescopes, notably the Atacama Large Millimeter/submillimeter Array in Chile’s Atacama Desert, has provided beautiful images of protoplanetary disks and maps of their chemical composition. This information is inspiring better models of how planets assemble from the dust and gases of a disk. Within our own solar system, the Rosetta mission has visited a comet, and OSIRIS-REx will visit, and even try to return samples from, an asteroid, which might give us the essential inventory of the materials that came together in our planet.
Once a planet like our Earth—not too hot and not too cold, not too dry and not too wet—has formed, what chemistry must develop to yield the building blocks of life? In the 1950s the iconic Miller-Urey experiment, which zapped a mixture of water and simple chemicals with electric pulses (to simulate the impact of lightning), demonstrated that amino acids, the building blocks of proteins, are easy to make. Other molecules of life turned out to be harder to synthesize, however, and it is now apparent that we need to completely reimagine the path from chemistry to life. The central reason hinges on the versatility of RNA, a very long molecule that plays a multitude of essential roles in all existing forms of life. RNA can not only act like an enzyme, it can also store and transmit information. Remarkably, all the protein in all organisms is made by the catalytic activity of the RNA component of the ribosome, the cellular machine that reads genetic information and makes protein molecules. This observation suggests that RNA dominated an early stage in the evolution of life.
Today the question of how chemistry on the infant Earth gave rise to RNA and to RNA-based cells is the central question of origin-of-life research. Some scientists think that life originally used simpler molecules and only later evolved RNA. Other researchers, however, are tackling the origin of RNA head-on, and exciting new ideas are revolutionizing this once quiet backwater of chemical research. Favored geochemical scenarios involve volcanic regions or impact craters, with complex organic chemistry, multiple sources of energy, and dynamic light-dark, hot-cold and wet-dry cycles. Strikingly, many of the chemical intermediates on the way to RNA crystallize out of reaction mixtures, self-purifying and potentially accumulating on the early Earth as organic minerals—reservoirs of material waiting to come to life when conditions change.
Assuming that key problem is solved, we will still need to understand how RNA was replicated within the first primitive cells. Researchers are just beginning to identify the sources of chemical energy that could enable the RNA to copy itself, but much remains to be done. If these hurdles can also be overcome, we may be able to build replicating, evolving RNA-based cells in the laboratory—recapitulating a possible route to the origin of life.
What next? Chemists are already asking whether our kind of life can be generated only through a single plausible pathway or whether multiple routes might lead from simple chemistry to RNA-based life and on to modern biology. Others are exploring variations on the chemistry of life, seeking clues as to the possible diversity of life “out there” in the universe. If all goes well, we will eventually learn how robust the transition from chemistry to biology is and therefore whether the universe is full of life-forms or—but for us—sterile.
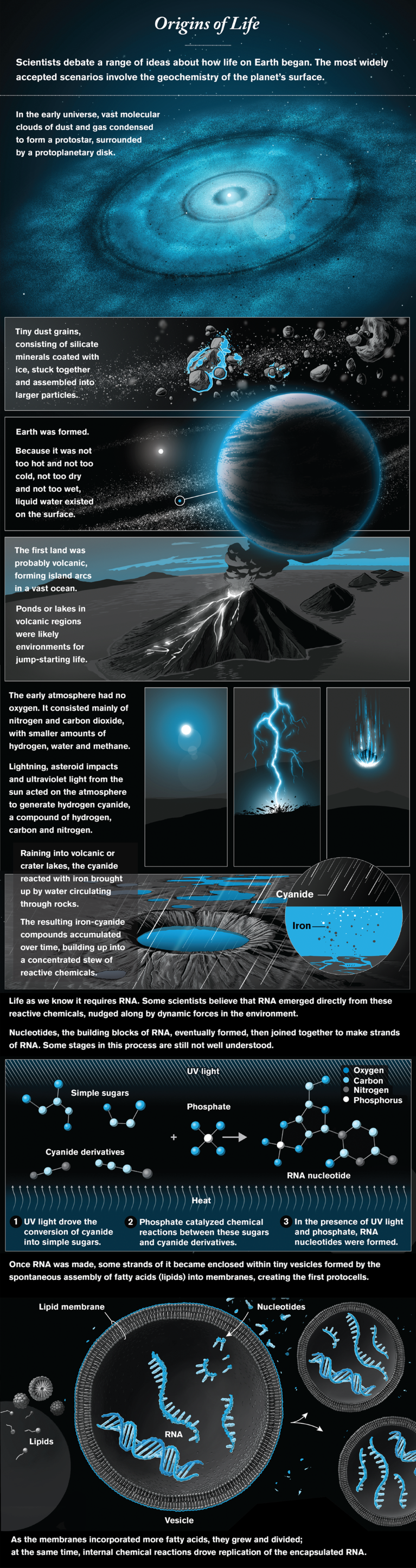
Illustration by Matthew Twombly
Nature 557 , S13-S15 (2018)
doi: https://doi.org/10.1038/d41586-018-05098-w
This article is part of Innovations In The Biggest Questions In Science , an editorially independent supplement produced with the financial support of third parties. About this content .
Related Articles
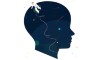
- Nanoscience and technology
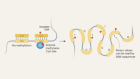
‘Do-it-yourself’ data storage on DNA paves way to simple archiving system
News & Views 23 OCT 24
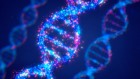
DNA stores data in bits after epigenetic upgrade
News 23 OCT 24
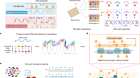
Parallel molecular data storage by printing epigenetic bits on DNA
Article 23 OCT 24
Assistant Professor of Molecular Genetics and Microbiology
The Department of Molecular Genetics and Microbiology at the University of New Mexico School of Medicine (http://mgm.unm.edu/index.html) is seeking...
University of New Mexico, Albuquerque
Assistant/Associate/Professor of Pediatrics (Neonatology)
Join the Department of Pediatrics at the University of Illinois College of Medicine Peoria as a full-time Neonatologist.
Peoria, Illinois
University of Illinois College of Medicine Peoria
Professor / Assistant Professor (Tenure Track) of Quantum Correlated Condensed and Synthetic Matter
The Department of Physics (www.phys.ethz.ch) at ETH Zurich invites applications for the above-mentioned position.
Zurich city
Associate or Senior Editor, Nature Communications (Structural Biology, Biochemistry, or Biophysics)
Job Title: Associate or Senior Editor, Nature Communications (Structural Biology, Biochemistry, or Biophysics) Locations: New York, Philadelphia, S...
New York City, New York (US)
Springer Nature Ltd
Faculty (Open Rank) - Bioengineering and Immunoengineering
The Pritzker School of Molecular Engineering (PME) at the University of Chicago invites applications for multiple faculty positions (open rank) in ...
Chicago, Illinois
University of Chicago, Pritzker School of Molecular Engineering
Sign up for the Nature Briefing newsletter — what matters in science, free to your inbox daily.
Quick links
- Explore articles by subject
- Guide to authors
- Editorial policies
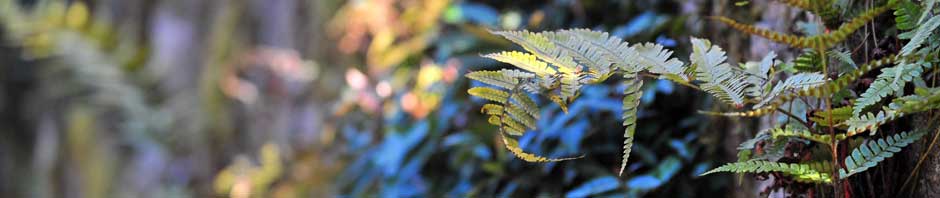
- Earth’s processes generate ecological patterns
- Behavioral Ecology
- Population Growth Models
- Survival and Reproduction at the Population Level
- Resources and Competition
- Predation and Community Diversity
- Food webs and Primary Production
- Energy Flow and Nutrient Cycles
- Strong Inference
- What is life?
- What is evolution?
- Evolution by Natural Selection
- Other Mechanisms of Evolution
- Population Genetics: the Hardy-Weinberg Principle
- Phylogenetic Trees
- Earth History and History of Life on Earth
Origin of Life on Earth
- Gene expression: DNA to protein
- Gene regulation
- Cell division: mitosis and meiosis
- Mendelian Genetics
- Chromosome theory of inheritance
- Patterns of inheritance
- Chemical context for biology: origin of life and chemical evolution
- Biological molecules
- Membranes and Transport
- Energy and enzymes
- Respiration, chemiosmosis and oxidative phosphorylation
- Oxidative pathways: electrons from food to electron carriers
- Fermentation, mitochondria, and regulation
- Why are plants green, and how did chlorophyll take over the world? (Converting light energy into chemical energy)
- Carbon fixation
- Recombinant DNA
- Cloning and Stem Cells
- Adaptive Immunity
- Human evolution and adaptation
Learning Objectives
- List and describe the four requirements for the origin of life on Earth
- Describe the four steps of the Oparin-Haldane hypothesis for the origin of life on Earth
- Cite evidence that all life on earth has a common origin
- Explain why RNA is hypothesized to be the first genetic material
- Apply the principles of evolution by natural selection to pre-biotic scenarios
- Use the principle of parsimony to infer the traits present in Last Universal Common Ancestor (LUCA)
The origin of life is a mystery, the ultimate chicken-and-egg conundrum ( Service 2015 ). When you and fellow students together discussed the defining characteristics of life , you probably included reproduction and hereditary information, transformation of energy, growth and response to the environment. You may also have said that, at least on Earth, all life is composed of cells, with membranes that form boundaries between the cell and its environment, and that cells were composed of organic molecules (composed of carbon, hydrogen, nitrogen, oxygen, phosphate, and sulfur – CHNOPS). The conundrum is that, on Earth today, all life comes from pre-existing life. Pasteur’s experiments disproved spontaneous generation of microbial life from boiled nutrient broth. No scientist has yet been able to create a living cell from organic molecules. So how could life have arisen on Earth, around 3.8 billion years ago ? (Keep in mind the scale of time we’re talking about here – the Earth is 4.6 billion years old, so it took almost a billion years for chemical evolution to result in biological life.) How can this question be addressed using the process of scientific inquiry?
Origin of life studies
Although scientists cannot directly address how life on Earth arose, they can formulate and test hypotheses about natural processes that could account for various intermediate steps, consistent with the geological evidence. In the 1920s, Alexander Oparin and J. B. S. Haldane independently proposed nearly identical hypotheses for how life originated on Earth. Their hypothesis is now called the Oparin-Haldane hypothesis, and the key steps are:
- formation of organic molecules, the building blocks of cells (e.g., amino acids, nucleotides, simple sugars)
- formation of polymers (longer chains) of organic molecules, that can function as enzymes to carry out metabolic reactions and possibly replicate (e.g., proteins, RNA strands),
- encoding hereditary information (RNA strands)
- formation of protocells; concentrations of organic molecules and polymers that carry out metabolic reactions within an enclosed system, separated from the environment by a semi-permeable membrane, such as a lipid bilayer membrane
The Oparin-Haldane hypothesis has been continually tested and revised, and any hypothesis about how life began must account for the primary universal requirements for life: the ability to reproduce, replication of hereditary information, the enclosure in membranes to form cells, and the use of energy to accomplish growth and reproduction.
1. How did organic molecules form on a pre-biotic Earth?
Miller-urey experiment.
Stanley Miller and Harold Urey tested the first step of the Oparin-Haldane hypothesis by investigating the formation of organic molecules from inorganic compounds. Their 1950s experiment produced a number of organic molecules, including amino acids, that are made and used by living cells to grow and replicate.
Miller and Urey used an experimental setup to recreate what environmental conditions were believed to be like on early Earth. A gaseous chamber simulated an atmosphere with reducing compounds (electron donors) such as methane, ammonia and hydrogen. Electrical sparks simulated lightning to provide energy. In only about a week’s time, this simple apparatus caused chemical reactions that produced a variety of organic molecules, some of which are the basic building blocks of life, such as amino acids. Although scientists no longer believe that pre-biotic Earth had an extremely reducing atmosphere, such reducing environments may be found in deep-sea hydrothermal vents, which also have a source of energy in the form of the heat from the vents. In addition, more recent experiments—that used conditions that are thought to better reflect the conditions of early Earth—have also produced a variety of organic molecules including amino acids and nucleotides (the building blocks of RNA and DNA) ( McCollom 2013 ).
The video below gives a nice overview of the rationale, setup, and findings from the Miller-Urey experiment (although it incorrectly overstates that Darwin showed that relatively simple creatures can gradually give rise to more complex creatures).
Organic molecules from meteors
Each day the Earth is bombarded with meteorites and dust from comets. Analyses of space dust and meteors that have landed on Earth have revealed that they contain many organic molecules. The in-fall of cometary dust and meteorites was far greater when the Earth was young (4 billion years ago). Many scientists believe that such extra-terrestrial organic matter contributed significantly to the organic molecules available at the time that life on Earth began. Scientists currently hypothesize three major sources of organic molecules on pre-life Earth: atmospheric synthesis by Miller-Urey chemistry, synthesis at deep-sea hydrothermal vents, and in-fall of organic molecules synthesized in outer space ( Bernstein 2006 ).
2. Formation of organic polymers
Given a high enough concentration of these basic organic molecules, under certain conditions these will link together to form polymers (chains of molecules covalently bonded together). For example, amino acids link together to form polypeptide chains, and the chains fold to become protein molecules. Ribose, a 5-carbon sugar, can bond with a nitrogenous base and phosphate to form a nucleotide. Nucleotides link together to form nucleic acids, like DNA and RNA. While this is accomplished now by enzymes in living cells, polymerization of organic molecules can also be catalyzed by certain types of clay or other types of mineral surfaces. Experiments testing this model have produced RNA molecules up to 50-units long, in only a 1-2 week period of time ( Ferris, 2006 ).
Enzymatic activity and hereditary information in one polymer: the RNA World hypothesis
The discovery by Thomas Cech that some RNA molecules can catalyze their own site-specific cleavage led to a Nobel prize (for Cech and Altman), the term ribozymes to denote catalytic RNA molecules, and the revival of a hypothesis that RNA molecules were the original hereditary molecules, pre-dating DNA in the information storage role. For origin-of-life researchers, here was the possibility that RNA molecules could both encode hereditary information and catalyze their own replication. DNA as the first hereditary molecule posed real problems for origin-of-life researchers because DNA replication requires protein enzymes (DNA polymerases) and RNA primers (see page on DNA replication), so it is difficult to envision how such a complex hereditary system could have evolved from scratch. With catalytic RNA molecules, a single molecule or family of similar molecules could potentially store genetic information and replicate themselves, with no proteins needed initially.
Populations of such catalytic RNA molecules would undergo a molecular evolution conceptually identical to biological evolution by natural selection. RNA molecules would make copies of each other, making mistakes and generating variants. The variants that were most successful at replicating themselves (recognize identical or very similar RNA molecules and most efficiently replicate them) would increase in frequency in the population of catalytic RNA molecules. The RNA world hypothesis envisions a stage in the origin of life where self-replicating RNA molecules eventually led to the evolution of a hereditary system in the first cells or protocells. A system of RNA molecules that encode codons to specify amino acids, and tRNA-like molecules conveying matching amino acids, and catalytic RNAs that create peptide bonds, would constitute a hereditary system much like today’s cells, without DNA.
At some point in the lineage leading to the Last Universal Common Ancestor, DNA became the preferred long-term storage molecule for genetic information. DNA molecules are more chemically stable than RNA (deoxyribose is more chemically inert than ribose). Having two complementary strands means that each strand of DNA can serve as a template for replication of its partner strand, providing some innate redundancy. These and possibly other traits gave cells with a DNA hereditary system a selective advantage so that all cellular life on Earth uses DNA to store and transmit genetic information.
Still, even today, ribozymes play universal and central roles in cellular information processing. The ribosome is a large complex of RNAs and proteins that reads the genetic information in a strand of RNA to synthesize proteins. The key catalytic activity, the formation of peptide bonds to link two amino acids together, is catalyzed by a ribosomal RNA molecule. The ribosome is a giant ribozyme. Since ribosomes are universal to all cells, such catalytic RNAs must have been present in the Last Universal Common Ancestor of all current life on Earth.
Visit the http://exploringorigins.org/ribozymes.html page to view the first ribozyme from Tetrahymena, discovered by Tom Cech, and the structure of the ribosomal RNAs.
The http://exploringorigins.org/nucleicacids.html page has videos of polymerization of RNA from nucleotides, template-directed RNA synthesis, and a model of RNA self-replication.
The video below explains the rationale behind the RNA world hypothesis and briefly describes some of the findings from different RNA world experiments.
3. Protocells: self-replicating and metabolic enzymes in a bag
All life on Earth is composed of cells. Cells have lipid membranes that separate their inner contents, the cytoplasm, from the environment. The lipid membranes allow cells to maintain high concentrations of molecules like nucleotides needed for self-replicating RNAs to function more efficiently. Cells also maintain large differences in concentration (concentration gradients) of ions across the membrane to drive transport processes and cellular energy metabolism.
Lipids are hydrophobic, and will spontaneously self-assemble in water to form either micelles or lipid bilayer vesicles. Vesicles that enclose self-replicating RNAs and other enzymes, take in reactants across the membrane, export products, grow by accretion of lipid micelles, and divide by fission of the vesicle, are called protocells or protobionts and may have been the precursors of cellular life.
See http://exploringorigins.org/protocells.html for video animations of protocells.
The video below explores the differences between chemical and biological evolution, and highlights protocells as an example of chemical evolution.
At what point would evolutionary processes, such as natural selection, begin to drive the origin of the first cells?
Biological evolution is restricted to living organisms. So once the first cells, complete with a hereditary system, were formed, they would be subject to evolutionary processes, and natural selection would drive adaptation to their local environments, and populations in different environments would undergo speciation as gene flow becomes restricted between isolated populations.
However, the RNA World Hypothesis envisions evolutionary processes driving populations of self-replicating RNA molecules or protocells containing such RNA molecules. RNA molecules that replicated imperfectly would produce daughter molecules with slightly different sequences. The ones that replicate better, or improve the growth replication of their host protocells, would have more progeny. Hence, molecular evolution of self-replicating RNA molecules or protocell populations containing self-replicating RNA molecules would favor the eventual formation of the first cells.
References and Resources
Patel et al. 2015 Common origins of RNA, protein and lipid precursors in a cyanosulfidic protometabolism. Nature Chemistry 7:301. DOI: 10.1038/NCHEM.2202 (with Science News article by R. Service ).
Bernstein M 2006. Prebiotic materials from on and off the early Earth. Philos Trans R Soc Lond B Biol Sci. 361:1689-700; discussion 1700-2. PubMed PMID: 17008210 ; PubMed Central PMCID: PMC1664678 .
Exploring Life’s Origins: http://exploringorigins.org/index.html
UN Sustainable Development Goal

SDG 3: Good Health and Well-being – The origin of life and the steps leading up to it are important topics of research in the field of astrobiology, which seeks to understand the conditions necessary for the emergence of life. Understanding the origin of life can provide insight into the fundamental processes that govern the development and evolution of living organisms, which can have implications for human health and well-being. For example, research into the origin of life and evolutionarily novel traits can lead to the development of new treatments for diseases, as well as the creation of new medicines and therapies.
4 Responses to Origin of Life on Earth

Here’s a link to a fun article on work by Georgia Tech’s Nick Hud using a $5 toaster oven for his origin-of-life research! http://nautil.us/issue/27/dark-matter/the-dawn-of-life-in-a-5-toaster-oven Thanks to Timothy for the find and the link
New paper reveals likely traits of the Last Universal Common Ancestor of all extant life: Weiss et al. 2016: The physiology and habitat of the last universal common ancestor, Nature Microbiology 1, Article number: 16116 doi:10.1038/nmicrobiol.2016.116 James McInerney wrote a brief commentary for the above article: http://www.nature.com/articles/nmicrobiol2016139
Fantastic (somewhat long) retelling of origin-of-life research, with the twist and turns, culminating with the current integrated hypotheses. http://www.bbc.com/earth/story/20161026-the-secret-of-how-life-on-earth-began
A new paper going beyond the Miller-Urey types of expts to explore what could emerge out of these prebiotic chemistries: Wolos et al 2020, Synthetic connectivity, emergence, and self-regeneration in the network of prebiotic chemistry https://science.sciencemag.org/content/369/6511/eaaw1955
Leave a Reply Cancel reply
You must be logged in to post a comment.
- Entries RSS
- Comments RSS
- Sites@GeorgiaTech
- Search for:
Creative Commons License

- eradication of Asian tiger mosquitos
- gut bacteria and stunted growth
- gut pathogens and autoimmune disease
- human milk oligosaccharides
- Legionella and mitochondria
- mannosides and uropathogenic E. coli
- origin of sickle cell mutation
- trehalose and C. difficile
- Uncategorized

IMAGES
VIDEO
COMMENTS
The origin of life on Earth stands as one of the great mysteries of science. Various answers have been proposed, all of which remain unverified. To find out if we are alone in the galaxy, we will need to better understand what geochemical conditions nurtured the first life forms.
Abstract. The origin of life (OOL) problem remains one of the more challenging scientific questions of all time. In this essay, we propose that following recent experimental and theoretical advances in systems chemistry, the underlying principle governing the emergence of life on the Earth can in its broadest sense be specified, and may be ...
Swedish chemist Svante A. Arrhenius suggested that life on Earth arose from “panspermia,” microscopic spores that wafted through space from planet to planet or solar system to solar system by radiation pressure. This idea, of course, avoids rather than solves the problem of the origin of life.
The history of life on Earth traces the processes by which living and extinct organisms evolved, from the earliest emergence of life to the present day.
The Origin of Life on Earth. Fresh clues hint at how the first living organisms arose from inanimate matter. By Alonso Ricardo & Jack W. Szostak. September 2009 Issue. The Sciences....
Today the question of how chemistry on the infant Earth gave rise to RNA and to RNA-based cells is the central question of origin-of-life research. Some scientists think that life...
Structures of communities of microorganisms, layered rocks called stromatolites, are found from more than three billion years ago. Since Earth is about 4.6 billion years old, these finds suggest that the origin of life must have occurred within a few hundred million years of that time.
Origin and Evolution of Earth. Questions about the origins and nature of Earth have long preoccupied human thought and the scientific endeavor. Deciphering the planet’s history and processes could improve the abil-ity to predict catastrophes like earthquakes and volcanoes, to manage Earth’s resources, and to anticipate changes in climate ...
List and describe the four requirements for the origin of life on Earth; Describe the four steps of the Oparin-Haldane hypothesis for the origin of life on Earth; Cite evidence that all life on earth has a common origin; Explain why RNA is hypothesized to be the first genetic material
origins of life studies and explores the development of the field to contextualize how it has evolved in response to discoveries in other fields. There is currently a great deal of argument regarding how life began on Earth, and how life may originate in general. Most professional scientists agree that there has not been to date an observed