Europe PMC requires Javascript to function effectively.
Either your web browser doesn't support Javascript or it is currently turned off. In the latter case, please turn on Javascript support in your web browser and reload this page.

Search life-sciences literature (44,156,633 articles, preprints and more)
- Available from publisher site using DOI. A subscription may be required. Full text
- Citations & impact
- Similar Articles
A guide to antigen processing and presentation.
Author information, affiliations.
- Pishesha N 1
- Harmand TJ 1
- Ploegh HL 1
ORCIDs linked to this article
- Ploegh HL | 0000-0002-1090-6071
Nature reviews. Immunology , 13 Apr 2022 , 22(12): 751-764 https://doi.org/10.1038/s41577-022-00707-2 PMID: 35418563
Abstract
Full text links .
Read article at publisher's site: https://doi.org/10.1038/s41577-022-00707-2
References
Articles referenced by this article (156)
The known unknowns of antigen processing and presentation.
Vyas JM , Van der Veen AG , Ploegh HL
Nat Rev Immunol, (8):607-618 2008
MED: 18641646
Structure of the human MHC-I peptide-loading complex.
Blees A , Januliene D , Hofmann T , Koller N , Schmidt C , Trowitzsch S , Moeller A , Tampe R
Nature, (7681):525-528 2017
MED: 29107940
Multifunctional Chaperone and Quality Control Complexes in Adaptive Immunity.
Trowitzsch S , Tampe R
Annu Rev Biophys, 135-161 2020
MED: 32004089
Recent advances in antigen processing and presentation.
Nat Immunol, (10):1041-1048 2007
MED: 17878914
The T cell receptor: critical role of the membrane environment in receptor assembly and function.
Call ME , Wucherpfennig KW
Annu Rev Immunol, 101-125 2005
MED: 15771567
B cell immunobiology in disease: evolving concepts from the clinic.
Martin F , Chan AC
Annu Rev Immunol, 467-496 2006
MED: 16551256
Control of dendritic cell cross-presentation by the major histocompatibility complex class I cytoplasmic domain.
Lizee G , Basha G , Tiong J , Julien JP , Tian M , Biron KE , Jefferies WA
Nat Immunol, (11):1065-1073 2003
MED: 14566337
Antigen processing and immune regulation in the response to tumours.
Reeves E , James E
Immunology, (1):16-24 2016
MED: 27658710
Defining the role of the MHC in autoimmunity: a review and pooled analysis.
Fernando MM , Stevens CR , Walsh EC , De Jager PL , Goyette P , Plenge RM , Vyse TJ , Rioux JD
PLoS Genet, (4):e1000024 2008
MED: 18437207
Towards a systems understanding of MHC class I and MHC class II antigen presentation.
Neefjes J , Jongsma ML , Paul P , Bakke O
Nat Rev Immunol, (12):823-836 2011
MED: 22076556
Citations & impact
Impact metrics, citations of article over time, alternative metrics.

Article citations
Characteristics of norovirus capsid protein-specific cd8 + t-cell responses in previously infected individuals..
He T , Deng Y , Zhang F , Zhang J , Zhu L , Wang Q , Ning J , Wu H , Yuan H , Li B , Wu C
Virulence , 15(1):2360133, 29 May 2024
Cited by: 0 articles | PMID: 38803081 | PMCID: PMC11141469
Determining the toxicological effects of indoor air pollution on both a healthy and an inflammatory-comprised model of the alveolar epithelial barrier in vitro.
Meldrum K , Evans SJ , Burgum MJ , Doak SH , Clift MJD
Part Fibre Toxicol , 21(1):25, 17 May 2024
Cited by: 0 articles | PMID: 38760786
Chaperone-mediated MHC-I peptide exchange in antigen presentation.
Jiang J , Natarajan K , Margulies DH
IUCrJ , 11(pt 3):287-298, 01 May 2024
Cited by: 0 articles | PMID: 38656309 | PMCID: PMC11067752
Antigenic Peptide-Thioredoxin Fusion Chimeras for In Vitro Stimulus of CD4 + TCR + Jurkat T Cells.
Ishina IA , Zakharova MY , Kurbatskaia IN , Mamedov AE , Belogurov AA , Rubtsov YP , Gabibov AG
Dokl Biochem Biophys , 516(1):53-57, 03 May 2024
Cited by: 0 articles | PMID: 38700816
Preclinical evaluation of two phylogenetically distant arenavirus vectors for the development of novel immunotherapeutic combination strategies for cancer treatment.
Raguz J , Pinto C , Pölzlbauer T , Habbeddine M , Rosskopf S , Strauß J , Just V , Schmidt S , Bidet Huang K , Stemeseder F , Schippers T , Stewart E , Jez J , Berraondo P , Orlinger KK , Lauterbach H
J Immunother Cancer , 12(4):e008286, 17 Apr 2024
Cited by: 0 articles | PMID: 38631709 | PMCID: PMC11029282
Similar Articles
To arrive at the top five similar articles we use a word-weighted algorithm to compare words from the Title and Abstract of each citation.
Invariant chain as a vehicle to load antigenic peptides on human MHC class I for cytotoxic T-cell activation.
Wälchli S , Kumari S , Fallang LE , Sand KM , Yang W , Landsverk OJ , Bakke O , Olweus J , Gregers TF
Eur J Immunol , 44(3):774-784, 27 Dec 2013
Cited by: 16 articles | PMID: 24293164
Mechanisms of antigen presentation.
Clin Chem Lab Med , 37(3):179-186, 01 Mar 1999
Cited by: 3 articles | PMID: 10353458
Inhibition of the MHC class II antigen presentation pathway by human cytomegalovirus.
Johnson DC , Hegde NR
Curr Top Microbiol Immunol , 269:101-115, 01 Jan 2002
Cited by: 30 articles | PMID: 12224504
Presentation of a self-peptide for in vivo tolerance induction of CD4+ T cells is governed by a processing factor that maps to the class II region of the major histocompatibility complex locus.
Fedoseyeva EV , Tam RC , Orr PL , Garovoy MR , Benichou G
J Exp Med , 182(5):1481-1491, 01 Nov 1995
Cited by: 10 articles | PMID: 7595218 | PMCID: PMC2192203
Autophagy Beyond Intracellular MHC Class II Antigen Presentation.
Trends Immunol , 37(11):755-763, 22 Sep 2016
Cited by: 76 articles | PMID: 27667710
Europe PMC is part of the ELIXIR infrastructure

An official website of the United States government
The .gov means it’s official. Federal government websites often end in .gov or .mil. Before sharing sensitive information, make sure you’re on a federal government site.
The site is secure. The https:// ensures that you are connecting to the official website and that any information you provide is encrypted and transmitted securely.
- Publications
- Account settings
Preview improvements coming to the PMC website in October 2024. Learn More or Try it out now .
- Advanced Search
- Journal List

Genetics of antigen processing and presentation
Adrian kelly.
Department of Pathology, University of Cambridge, Cambridge, CB21QP UK
John Trowsdale
Immune response to disease requires coordinated expression of an army of molecules. The highly polymorphic MHC class I and class II molecules are key to control of specificity of antigen presentation. Processing of the antigen, to peptides or other moieties, requires other sets of molecules. For classical class I, this includes TAP peptide transporters, proteasome components and Tapasin, genes which are encoded within the MHC. Similarly, HLA-DO and -DM, which influence presentation by HLA class II molecules, are encoded in the MHC region. Analysis of MHC mutants, including point mutations and large deletions, has been central to understanding the roles of these genes. Mouse genetics has also played a major role. Many other genes have been identified including those controlling expression of HLA class I and class II at the transcriptional level. Another genetic approach that has provided insight has been the analysis of microorganisms, including viruses and bacteria that escape immune recognition by blocking these antigen processing and presentation pathways. Here, we provide a brief history of the genetic approaches, both traditional and modern, that have been used in the quest to understand antigen processing and presentation.
Some history
The early history of the genetics of antigen processing and presentation followed from the work on histocompatibility in mice, initially by Peter Gorer, who worked at the Lister Institute and Guy’s hospital in London. His discovery of the MHC in turn was inspired by three developments. First was the curious pastime of inbreeding mouse strains, a fashionable hobby which spread from China. It reached America in the early 1900s, and after a while, pioneering geneticists realised the advantage of inbred strains for research. Many of the mouse strains were started over 100 years ago. C57BL, one of the original strains, was designated as the mice were black and were number 57. BALB/c mice were white, on the other hand, and were designated by their originator, Halsey J. Bagg, as Bagg albino, or BALB/c for short. A fascinating early history of these developments is presented in a book on the natural history of the MHC (Klein 1986 ).
Inbred strains were pivotal in the next development, where researchers used them to study the genetics of tumour rejection. As early as 1903, it was discovered that tumours that grew well when transferred within the same strain were rejected in a different one. Then, in 1922, Little and Johnson showed that transplantation of normal tissue was subject to the same strain specificity as tumours. A third stimulus was the development of blood group research, largely attributed to Landsteiner.
JB Haldane suggested that tumour resistance factors may be akin to blood group antigens, but it was Gorer who performed experiments to test the idea that antigens were shared by both malignant and normal tissues. This led to the formulation of an immunological theory of transplantation, which was later systematised by Peter Medawar. George Snell was studying similar phenomena, and after collaborating with Gorer, he proposed calling the tumour-resistance factors Histocompatibility genes. His approach started to reveal some of the complexity of histocompatibility.
Early work leading to the discovery of the human HLA complex developed in the 1950s and was dependent on the study of antibodies against alloantigens on white blood cells by three laboratories: Jean Dausset in Paris, Rose Payne and Walter Bodmer in Stanford, and Jon van Rood in Leiden. It was realised that some patients, and women who had borne several children, tended to make such antibodies, which were independent of ABO blood groups and erythrocytes. At the time, human organ transplantation was becoming widespread, and it gradually became accepted that human leukocyte antigens were the equivalent of mouse H-2 antigens. The hope was that careful matching, as in ABO, could lead to organ transplants that were not rejected. It was soon realised that the HLA system was more complex than ABO and progress depended on exchange of cells and antisera. The International Histocompatibility Workshops , which have been held every few years since 1964, were critical in interpreting and integrating information obtained with a variety of techniques from different laboratories. Analysis of data from these workshops indicated that a single genetic region was pivotal, namely, in humans, HLA, the Major Histocompatibility Complex. An associated protein chain, β2microglobulin, was identified as a component of HLA antigens and was later mapped outside the complex to chromosome 15 (Goodfellow et al. 1975 ).
Attempts to develop in vitro assays to study graft rejection led to the mixed lymphocyte reaction (MLR), which also turned out to be controlled by the MHC region. However, the results did not correlate completely with the serologically defined determinants. Work in both human and mouse indicated that these determinants, as well as MLR responsiveness, were both part of the H2 and HLA complexes but were separated genetically. Meanwhile, a different set of experiments showed that levels of antibody response to short synthetic polypeptides were controlled by the MHC. For example, C57 mice responded well to the branched synthetic polypeptide (T, G)-A-L, but CBA animals were poor responders. These effects were traced to the H-2 region leading to the so-called immune response (Ir) genes (Benacerraf and McDevitt 1972 ).
These many years of work, all indicated that the MHC was a major hub controlling a number of immunological phenomena. Indeed, additional experiments showed that the MHC also controlled susceptibility to viruses. Work in Canberra showed that cytotoxic T cells simultaneously recognised viral antigens and MHC molecules, in a phenomenon that came to be known as MHC restriction (Zinkernagel and Doherty 1974 ).
The nature of the MHC became even more complex when it was proposed that T cell suppression was also controlled by the class II region (Green et al. 1983 ). There followed a decade of controversy over the existence of this phenomenon (Bloom et al. 1992 ). It was eventually accepted, and the responsible T cells were called regulatory to distinguish them from the confusing history of suppression (Sakaguchi et al. 2007 ). The genetics behind the controversy concerned the I-J gene which was supposed to map to the I region of the mouse MHC and control the function of suppressor T cells. The I-J antigens were proposed to be soluble molecules secreted by suppressor T cells. It was a shock to find that discrete I-J genes did not exist once the I region of the mouse had been cloned (Kronenberg et al. 1983 ).
The genetics of antigen processing and presentation was advanced dramatically in the late 1980s when two further major technical developments helped to get to grips with the complexity. One was the DNA cloning revolution and the second was the determination of the structure of MHC molecules from crystals. Initial cloning of H-2 and HLA antigen genes led quickly to assembly of maps of the MHC in humans and mice. These dramatically simplified the picture to just a handful of class I and class II loci, albeit with a profound level of polymorphism. The early maps of mouse MHC were painstakingly assembled from overlapping cosmids (Steinmetz and Hood 1983 ). Many different human haplotypes have been analysed by these techniques (Horton et al. 2008 ; Shiina et al. 2004 ), as well as using more modern, high-throughput approaches (Norman et al. 2016 ).
The nature of the polymorphism was mysterious, as class I and class II chains encompassed many amino acid changes seemingly scattered throughout the first two domains of class I and the first domains of both chains of class II. The breakthrough came with the crystal structure of the first MHC antigen, HLA-A2 by Pamela Bjorkman, who was a PhD student at the time in the Wiley/Strominger laboratories (Bjorkman et al. 1987a , Bjorkman et al. 1987b ). The realisation that class I and class II molecules possessed a groove which bound peptides immediately swept away other models of antigen recognition, such as those invoking independent receptors for antigen and histocompatibility molecules on T cells.
The development of molecular immunology through the creation of mutants, DNA sequencing, protein structure and gene discovery then paved the way for uncovering the various components of the antigen processing and presentation pathways, as outlined below (Table (Table1 1 ).
Some human antigen processing and presenting components. Alternative names and gene designations are given in parentheses
Mutant cell lines
Panels of HLA homozygous typing cell lines have been important tools in the analysis of human tissue types through HLA classes I and II. These lines were derived by Epstein Barr Virus transformation of lymphoid cells from consanguineous mating, usually first cousin. They have been used for over 40 years by the HLA community and are still in use today (Turner et al. 2018 ).
Analysis of the involvement of the HLA region in antigen processing and presentation was further enhanced by the generation of deletions and other mutations in lymphoblastoid cell lines (Fig. (Fig.1). 1 ). One series of mutants was made by γ ray mutagenesis of the cell line B-LCL721 by the DeMars group (DeMars et al. 1984 ; Kavathas et al. 1980 ). Cells were subjected to 2 cycles of mutagenesis followed by immune-selection with monoclonal antibodies for MHC antigen loss. B-LCL721 contains the following two MHC haplotypes:
- HLA-A*01 HLA-B*08 HLA-DRB1*03 HLA-DPB1*04
- HLA-A*02 HLA-B*51 HLA-DRB1*01 HLA-DPB1*02

Composition of the mutant lines derived in the DeMars laboratory (DeMars et al. 1984 ; Kavathas et al. 1980 ) and the further derivative T2 (Salter et al. 1985 ). The shaded boxes show the proposed extents of the deletions. See text for more details of typing. These mutant cells have been used in numerous antigen processing and presentation studies. The Figure adapted from Demars et al. 1984
More details on the class I HLA types are available on the IPD-IMGT/HLA database. 1 To our knowledge, not all class II genes have been typed in these cells. Mutant cell lines were isolated by subjecting these cells to irradiation. After 5 days growth, the survivors were selected with complement and a variety of monoclonal antibodies. Analysis of these mutants showed that they contained large homozygous or hemizygous deletions over the MHC region (Fig. 1 ). One such line LCL721.174, and its derivative 174xCEM.T2, has been used extensively to investigate TAP transporter function and MHC class I peptide loading. 174xCEM.T2, more commonly referred to as T2, was generated by fusing LCL721.174 with CEMR.3, a T-LCL, and selecting for loss of CEMR.3-derived copies of chromosome 6 (Salter et al. 1985 ). Both 174XCEM.T2 and LCL721.174 therefore carry the same chromosome 6 MHC deletion.
A similar procedure was used by Andreas Ziegler, using the lymphoma cell line BJAB.B95.8.6 (Spring et al. 1985 ). The wild-type cells were typed with the resolution available at that time as:
- HLA-A*1 HLA-B*35 HLA-C*4 HLA-DR*5 HLA-DQw3 HLA-DP*4
- HLA-A*2 HLA-B*13 HLA-C* HLA-DR* HLA-DQw1 HLA-DP*2
The Pious laboratory used a variation of this approach to isolate mutants in class II (8.1.6 and 9.28.6) by mutagenizing with EMS an HLA-DR3+ cell line, T5-1 (Mellins et al. 1988 ; Pious et al. 1985 ). Similarly, in mouse studies designed to investigate the nature of self and non-self-recognition, Klaus Karre generated a murine cell line RMA-S that lacked surface H-2 expression but was rejected in tumour transplant models (Karre et al. 1986 ). Analysis of this cell line was instrumental in providing early models for peptide loading by MHC class I molecules (Townsend et al. 1989 ). In addition, Roberto Accolla irradiated Raji cells to produce a mutant line RJ2.2.5 selecting against HLA-DR expression with an antibody D1–12(Accolla 1983 ), facilitating identification of CIITA.
These mutant cell lines and their derivatives have been instrumental in a long series of experimental approaches to uncover various components of antigen processing, and they continue to be important tools in investigating their mechanism of action.
TAP transporters
A key insight into antigen processing was the finding that influenza A-specific cytotoxic T lymphocytes (CTL) recognise discrete epitopes of the nucleoprotein molecule. These data implied a mechanism for generation of viral protein fragments and their transport to the cell surface for presentation to CTL (Townsend et al. 1985 ). The peptide-binding groove in HLA molecules was a strong contender for the peptide carrier. There was a problem though in that MHC molecules were integrated into the cell membrane, so how did peptide fragments, which lacked signal sequences, access the endoplasmic reticulum (ER) to be loaded? How were the peptides transported to the cell surface on MHC molecules?
The solution came from MHC mapping and analysis of the mutants referred to above. Several groups simultaneously identified genes encoding transporter molecules mapping to the MHC. These were transporters of the so-called ABC (ATP-binding cassette) type, which were subsequently named TAP, for Transporters Associated with Antigen Processing (Deverson et al. 1990 ; Monaco et al. 1990 ; Spies et al. 1990 ; Trowsdale et al. 1990 ). These data suggested immediately that the transporters, encoded as a heterodimer of two proteins, TAP1 and TAP2, were responsible for pumping peptides into the ER (Kelly et al. 1992 ). To confirm this, the TAP1 sequence restored HLA class I expression when transfected into a cell line with a mutant TAP1 gene, LCL721.134 (Spies and DeMars 1991 ), and the TAP2 sequence restored function and co-precipitation of TAP1 and TAP2 when transfected into cells deficient in TAP2 (Kelly et al. 1992 ). However, this was not the case when using LCL721.174 cells, which maintained a large deletion over the class II region, covering both components of the heterodimer, TAP1 and TAP2(Spies and DeMars 1991 ). Studies of rats and chickens were particularly informative as the TAP genes are polymorphic in these species. It turned out that specific allelic versions of TAP supply peptides appropriate for class I molecules whose genes are linked in cis on the haplotype. Inappropriate pairing of TAP alleles with class I alleles they serve with non-binding peptides led to reduced expression of class I, which could be detected at the cell surface (Deverson et al. 1990 ; Kaufman 2015 ; Powis et al. 1996 ).
Natural mutants in TAP
A number of individuals have been identified with immune-deficiencies due to mutations in TAP (de la Salle et al. 1994 ; Zimmer et al. 2005 ). These belong to the category of type I bare lymphocyte (BLS) syndrome, where HLA class I, but not class II, is affected. These patients generally have a severe reduction of class I at the cell surface, but in spite of this, most of these individuals reach adulthood. They are rare and are almost exclusively HLA homozygous due to first cousin parentage. They generally display chronic infections of the respiratory tract (purulent rhinitis, pansinusitis, otitis media) with bacteria ( H. influenzae , S. pneumoniae , S. aureus , K. pneumoniae , E. coli and P. aeruginosa ). About half of them exhibit granulomatous skin lesions. This may be due to suboptimal cytokine and cellular responses, leading to tissue damage and favouring subsequent bacterial infection and further recruitment of phagocytic cells. Indeed, antibiotics that impair neutrophils appear to be beneficial.
Patients with defects in TAP do not suffer from severe viral infection. This is unexpected but may relate to the fact that they have a normal humoral (antibody) response and significant numbers of T cells, particularly γδT cells, as well as NK cells and neutrophils. Additionally, presentation of some viral antigens is TAP-independent. Another symptom in these patients is necrotizing granulomatous lesions particularly around the nose, resulting in loss of the septum and associated cartilage. Presentation is variable though. The skin ulcers were found to contain macrophages and NK cells.
In terms of biological effects, the proportion of T cells may be reduced in patients with TAP defects, but there is wide variation in phenotype. They tend to have a higher proportion of γδT cells. Interestingly, numbers of NK cells are normal, but they have no cytotoxic activity against the standard class I-negative targets, unless activated by cytokines.
Proteasome components—LMPs
At the same time that TAPs were discovered, clues to the proteolytic machinery, responsible for breaking the antigenic proteins into peptides, were also provided, by studying mutants over the MHC region. The biochemical prelude to this discovery came in the early 1980s when Monaco and McDevitt described a series of up to16 low-molecular weight proteins , LMPs, that mapped to the MHC. Initially, the functions of these proteins were not obvious, but they formed a complex by co-precipitation and varied between different mouse haplotypes, in other words were polymorphic (Monaco and McDevitt 1984 ). It was subsequently established that genes for two interferon-inducible, catalytic proteasome subunits, LMP2 (PSMB9; β1i) and LMP7 (PSMB8: β5i), were closely linked to the TAP1 and TAP2 genes in both human and mouse MHC (Glynne et al. 1991 ; Kelly et al. 1991a ). As with the TAPs, finding proteasome components encoded in the MHC immediately suggested that the proteasome was responsible for producing the antigenic peptides. Proteasomes are ubiquitous cellular components responsible for continual turnover of proteins in general. However, the MHC-encoded proteasome genes were interferon inducible and, when expressed, replaced constitutive components in a subset of the main structures. Another interferon-inducible subunit PSMB10 (β2i) is encoded outside the MHC on chromosome 16q22.1.
ERp57 and ERAP1
A number of chaperones are associated with class I as it matures and picks up peptide in the peptide-loading complex. These chaperones serve multiple proteins in the ER and are not dedicated to class I. However, ERp57 is a component of the peptide-loading complex. The protein aids folding of nascent glycoproteins by facilitating disulphide bond isomerisation.
ERAP1 is an amino peptidase. It plays a role in determining the length and sequence of peptides bound and presented by class I allotypes. HLA-B27 is strongly associated with ankylosing spondylitis (Chen et al. 2014 ), and genetic studies indicated that certain ERAP1 allotypes are more associated with ankylosing spondylitis than others, due to their contribution to peptide trimming (Reeves et al. 2014a , Reeves et al. 2014b ). There has been some controversy over this effect as it was difficult to analyse genetically. It was proposed to involve specific combinations of different ERAP1 allotypes, making association studies difficult (Reeves et al. 2015 ; Robinson and Brown 2015 ).
The gene for Tapasin, another component of the antigen processing machinery, is located just outside the MHC region (Ortmann et al. 1997 ). The product of this gene, which is an additional member of the immunoglobulin gene family, links the peptide transporter to the nascent class I molecule in a complex, the peptide-loading complex (Blees et al. 2017 ). Optimisation of the MHC class I peptide cargo is proposed to be dependent on Tapasin (Williams et al. 2002 ). However, class I molecules vary in their dependence on the protein, an effect that has been mapped to specific positions on the HLA molecule (Park et al. 2003 ) as well as to its conformational flexibility (Garstka et al. 2011 ). For example, the HLA-B*44 allele, which differs exclusively at position 156, B*44:02 (156Asp), is Tapasin-dependent, whereas other alleles are not (Badrinath et al. 2012 ). The reason for this is not known but could relate to the fact that some virus products compromise antigen presentation by interacting with the Tapasin protein.
There is evidence that the jawed vertebrate genome has undergone duplication at least twice in its evolutionary history, according to the 2R hypothesis. Accordingly, it was demonstrated that there are traces of at least four clusters of genes related to those in the MHC proper on chromosome 6 (Kasahara 1999 ). One paralogous region, identified by Louis Du Pasquier, was at chromosome position 12p13.3. A gene in this region shared sequence homology with Tapasin, dubbed TAPBPR (Teng et al. 2002 ). The product of this gene was subsequently shown to be a novel component of the MHC class I presentation pathway, which acts as a peptide exchange catalyst (Hermann et al. 2015 ; Neerincx and Boyle 2017 ). TAPBPR interacts with class I in a similar manner to Tapasin but is not complexed to TAP (Jiang et al. 2017 ). TAPBPR links UDP-glucose:glycoprotein glucosyltransferase 1 onto MHC class I (Neerincx et al. 2017 ). Tapasin is monomorphic, but TAPBPR is relatively polymorphic and there are a number of common alleles and splice variants in humans (Porter et al. 2014 ). The relationship between the products of these alleles and MHC Class I loci and alleles is not known. As with Tapasin, it appears that some alleles of class I are dependent, and others independent, of the molecule.
HLA-DM and DO
The existence of an MHC-encoded factor that was essential for MHC class II antigen presentation was first suggested through the characterisation of mutagenised B lymphoblastoid cells selected for loss of HLA-DR3 reactivity with a DR3-specific antibody, 16:23(Mellins et al. 1990 ). These cells had normal levels of cell surface DR but were unable to present native antigen to T cells. They possessed class II molecules that were predominantly filled with the CLIP peptide and fell apart in the presence of SDS. The defect was eventually mapped to HLA-DM (Morris et al. 1994 ), a class II-related molecule previously identified in both mouse and human in screens for expressed genes encoded on cosmids spanning the MHC region (Kelly et al. 1991b ; Cho et al. 1991 ). HLA-DM acts as a peptide editing chaperone stabilising class II whilst exchanging CLIP for antigenic peptide. DM activity is regulated by a second non-classical class II molecule, now called HLA-DO. HLA-DO alpha and beta chains were first identified due to their high homology with human and murine classical class II molecules (Larhammar et al. 1985 ; Tonnelle et al. 1985 ; Trowsdale and Kelly 1985 ). It proved much harder to decipher the role of DO even though the molecule was identified some 6 years before DM. DOA and DOB were not located together in the MHC, and the chains showed different mRNA expression patterns initially suggesting that they would not associate as a pair. DO resides in late endosomal compartments but requires association with DM in the endoplasmic reticulum for correct assembly (Liljedahl et al. 1996 ). The in vivo role of DO is still debatable, but mechanistically, it functions as a negative regulator of DM activity (van Ham et al. 1997 ; Denzin et al. 1997 ). Unlike the transient interactions seen between DM and DR, the DM/DO interaction is very stable, suggesting that DO acts as a competitive inhibitor of DM, a view consistent with the DM/DO crystal structure (Guce et al. 2013 ).
Haplotypes and linkage disequilibrium
It has been known for some time that the MHC comprises a large region in linkage disequilibrium (LD), or polymorphic frozen blocks (Dawkins et al. 1999 ). In some populations, for example, combinations of alleles, such as HLA-A1-B8-DR3, are more commonly found together than would be expected based on frequencies of individual alleles in the population. Several mechanisms may be invoked to explain this. First, there may have been insufficient time for recombination after relatively recent expansion of families, in some cases in isolated populations. Another possibility is that recombination ‘cold spots’ in the MHC sequence restrain the level of exchange of alleles between haplotypes. This may be facilitated by a reduced level of pairing at meiosis in a region such as the MHC, where the variation between haplotypes compromises homologous interaction. Indeed, overall recombination is low in the MHC.
An attractive explanation for the high LD is clustering of alleles that encode proteins that work well together and maintenance of functionally coordinated sets of alleles. As discussed above, the discovery of both TAP transporters and LMP proteasome components mapping to the MHC was largely serendipitous and was inspired to some extent by the notion that the MHC was a cluster of genes with inter-related functions. Furthermore, it was proposed that linkage of polymorphic transporters with polymorphic MHC class I genes permitted functional coordination, such that appropriate binding peptides, for the class I molecules linked in cis , were pumped by the relevant TAP. This turned out to be the case for rats and chickens, where genes for class I and TAP are in close proximity (Powis et al. 1992 ; Tregaskes et al. 2016 ). In the human MHC TAP transporters, genes are separated from the class I genes they serve by the class III region, so this functional integration may not be fully operational in our species. Another consideration is that each individual has two haplotypes, the various components of which must cooperate to some extent.
Recent data add weight to the notion of a high degree of allelic integration on individual haplotypes. It is becoming appreciated that, in addition to protein-coding loci, other polymorphic genomic features are embedded in the MHC complex. One clue to this came from the finding that a single nucleotide polymorphism (SNP) 35 kb upstream of HLA-C associated with levels of mRNA transcript and cell-surface expression (Kulkarni et al. 2011 ). Binding of microRNA hsa-miR-148 to a variable 3′ untranslated region in HLA-C genes regulated their expression. The conclusion was that expression levels may be regulated both by cis- and trans- acting factors. A further indication of the integration along haplotypes came from data relating to the way that class I molecules ‘educate’ by interacting with receptors on NK cells. Hydrophobic leader sequences from class I molecules supply peptides that bind HLA-E, which instructs CD94/NKG2A receptors on NK cells. Leader sequences of HLA-B molecules are dimorphic: Those with − 21 methionine (− 21 M) provide peptides that bind HLA-E, whereas − 21 threonine do not. Those haplotypes with -21 M rarely encode the ligands for other NK receptors, namely Bw4+ HLA-B and C2+ HLA-C. From these data, it was proposed that there are two schools of HLA haplotypes; one focused on supplying CD94/NKG2A ligands, and the other, KIR ligands. Individuals with − 21 M had NKG2A+ cells that were more effective than those with only − 21 T (Horowitz et al. 2016 ). Similarly, HLA-A leader sequences determine expression levels of HLA-E, again influencing interaction with CD94/NKG2A on NK cells and in turn HIV replication (Ramsuran et al. 2018 ).
Regulation of transcription and translation
Analysis of mutants has also been productive for identifying regulators of antigen processing and presenting genes. For many years, it was difficult to track down a master regulator of class I. On the other hand, mutants affecting class II were isolated from bare lymphocyte patients (Reith and Mach 2001 ). The class II master regulator CIITA is a nucleotide-binding domain and leucine-rich repeat receptor (NLR) protein. This large gene was initially found by expression cloning in a class II-deficient cell line (Steimle et al. 1993 ). Mutants invoking loss of function of CIITA generally result in severe immunodeficiency, a form of bare lymphocyte syndrome. Individuals with BLS have impaired antibody and T cell responses due to the lack of MHC class II expression. Class II transcription requires, in addition to CIITA, at least three additional factors: RFX5, RFX-AP and RFX-ANK. The genes encoding these factors were identified by comparing panels of rare BLS patients and mutant cell lines selected for loss of class II expression. This identified four complementation groups. Transient heterokaryon fusions between these cell lines restored class II expression if the partners harboured defects in different components, thereby defining the four groups. A combination of genetic complementation and protein characterisation eventually identified the genetic lesions, as reviewed in Reith and Mach ( 2001 ).
It was only in the last decade that a master transcription regulator for class I was discovered (Kobayashi and Elsen, 2012 ; Meissner et al. 2010 ; Neerincx et al. 2013 ). Like the class II transcription activator, CIITA, NLRC5 is an NLR protein and the two molecules share some homology. In cell lines defective in class I expression, such as mouse melanoma B16F10, over-expressed NLRC5 could restore class I expression. In human cells, over-expression of NLRC5 resulted in induction of non-classical class I genes HLA-E, -F and -G, which normally exhibit a restricted tissue expression. CIITA-dependent activation of HLA class II requires the enhanceosome complex, which binds to a motif, SXY, in the promoter of the gene. NLRCF probably interacts with a similar module upstream of class I genes.
Confirmation of the role of NLRC5 in regulation of MHC class I was provided by studying Nlrc5 -deficient knock-out mice (Staehli et al. 2012 ). The data showed that MHC class I was downregulated, exhibiting the greatest effect in cells in the immune system. However, treatment of these mice with inflammatory stimuli, such as interferon or LPS, resulted in significant class I expression, indicating that Nlrc5 -independent mechanisms must exist to regulate MHC class I.
Recent work suggests that there is still more to learn about variation in HLA expression. For example, the Anderson laboratory identified an elaborate system regulating forms of HLA-C, which is specific to natural killer cells, and could relate to NK cell licencing or education (Li et al. 2018 ). Other work suggests that, in addition to allelic differences, levels of class I should be taken into account in relation to autoimmunity, infection and transplantation (Apps et al. 2013 ; Kaur et al. 2017 ; Li et al. 2018 ; Petersdorf et al. 2014 ).
Screening for novel components
New screening techniques have the potential to identify novel factors involved in MHC biology. For example, forward genetic screens involving retroviral insertional mutagenesis or random CRISPR/Cas9 targeting of a haploid human cell line KBM7 implicate TXNDC11 as a novel factor required for MHC class I endoplasmic reticulum-associated protein degradation (ERAD) (Timms et al. 2016 ). In addition, a genome-wide RNAi screen was used to identify pathways regulating MHC class II antigen presentation (Paul et al. 2011 ).
Immunogenetics has played a predominant role in uncovering many of the fundamentals of antigen processing and presentation. In this review, we have sketched out some of the history and background to the genetics, focussing mainly on the human system. Other contributions to this volume of Immunogenetics may be consulted for more in-depth coverage of individual components of antigen processing and presentation.
Funding information
JT is supported by the European Research Council under the European Union’s Horizon 2020 research and innovation programme (Grant agreement No. 695551).
1 https://www.ebi.ac.uk/cgi-bin/ipd/imgt/hla/fetch_cell.cgi?10972
This article is part of the Topical Collection on Biology and Evolution of Antigen Presentation
- Accolla RS. Human B cell variants immunoselected against a single Ia antigen subset have lost expression of several Ia antigen subsets. J Exp Med. 1983; 157 :1053–1058. doi: 10.1084/jem.157.3.1053. [ PMC free article ] [ PubMed ] [ CrossRef ] [ Google Scholar ]
- Apps R, Qi Y, Carlson JM, Chen H, Gao X, Thomas R, Yuki Y, Del Prete GQ, Goulder P, Brumme ZL, Brumme CJ, John M, Mallal S, Nelson G, Bosch R, Heckerman D, Stein JL, Soderberg KA, Moody MA, Denny TN, Zeng X, Fang J, Moffett A, Lifson JD, Goedert JJ, Buchbinder S, Kirk GD, Fellay J, McLaren P, Deeks SG, Pereyra F, Walker B, Michael NL, Weintrob A, Wolinsky S, Liao W, Carrington M. Influence of HLA-C expression level on HIV control. Science. 2013; 340 :87–91. doi: 10.1126/science.1232685. [ PMC free article ] [ PubMed ] [ CrossRef ] [ Google Scholar ]
- Badrinath S, Saunders P, Huyton T, Aufderbeck S, Hiller O, Blasczyk R, Bade-Doeding C. Position 156 influences the peptide repertoire and tapasin dependency of human leukocyte antigen B*44 allotypes. Haematologica. 2012; 97 :98–106. doi: 10.3324/haematol.2011.046037. [ PMC free article ] [ PubMed ] [ CrossRef ] [ Google Scholar ]
- Benacerraf B, McDevitt HO. Histocompatibility-linked immune response genes. Science. 1972; 175 :273–279. doi: 10.1126/science.175.4019.273. [ PubMed ] [ CrossRef ] [ Google Scholar ]
- Bjorkman PJ, Saper MA, Samraoui B, Bennett WS, Strominger JL, Wiley DC. The foreign antigen binding site and T cell recognition regions of class I histocompatibility antigens. Nature. 1987; 329 :512–518. doi: 10.1038/329512a0. [ PubMed ] [ CrossRef ] [ Google Scholar ]
- Bjorkman PJ, Saper MA, Samraoui B, Bennett WS, Strominger JL, Wiley DC. Structure of the human class I histocompatibility antigen, HLA-A2. Nature. 1987; 329 :506–512. doi: 10.1038/329506a0. [ PubMed ] [ CrossRef ] [ Google Scholar ]
- Blees A, Januliene D, Hofmann T, Koller N, Schmidt C, Trowitzsch S, Moeller A, Tampe R. Structure of the human MHC-I peptide-loading complex. Nature. 2017; 551 :525–528. doi: 10.1038/nature24627. [ PubMed ] [ CrossRef ] [ Google Scholar ]
- Bloom BR, Salgame P, Diamond B. Revisiting and revising suppressor T cells. Immunol Today. 1992; 13 :131–136. doi: 10.1016/0167-5699(92)90110-S. [ PubMed ] [ CrossRef ] [ Google Scholar ]
- Chen L, Fischer R, Peng Y, Reeves E, McHugh K, Ternette N, Hanke T, Dong T, Elliott T, Shastri N, Kollnberger S, James E, Kessler B, Bowness P. Critical role of endoplasmic reticulum aminopeptidase 1 in determining the length and sequence of peptides bound and presented by HLA-B27. Arthritis Rheumatol. 2014; 66 :284–294. doi: 10.1002/art.38249. [ PubMed ] [ CrossRef ] [ Google Scholar ]
- Cho SG, Attaya M, Monaco JJ. New class II-like genes in the murine MHC. Nature. 1991; 353 :573–576. doi: 10.1038/353573a0. [ PubMed ] [ CrossRef ] [ Google Scholar ]
- Dawkins R, Leelayuwat C, Gaudieri S, Tay G, Hui J, Cattley S, Martinez P, Kulski J. Genomics of the major histocompatibility complex: haplotypes, duplication, retroviruses and disease. Immunol Rev. 1999; 167 :275–304. doi: 10.1111/j.1600-065X.1999.tb01399.x. [ PubMed ] [ CrossRef ] [ Google Scholar ]
- de la Salle H, Hanau D, Fricker D, Urlacher A, Kelly A, Salamero J, Powis SH, Donato L, Bausinger H, Laforet M, et al. Homozygous human TAP peptide transporter mutation in HLA class I deficiency. Science. 1994; 265 :237–241. doi: 10.1126/science.7517574. [ PubMed ] [ CrossRef ] [ Google Scholar ]
- DeMars R, Chang CC, Shaw S, Reitnauer PJ, Sondel PM. Homozygous deletions that simultaneously eliminate expressions of class I and class II antigens of EBV-transformed B-lymphoblastoid cells. I. Reduced proliferative responses of autologous and allogeneic T cells to mutant cells that have decreased expression of class II antigens. Hum Immunol. 1984; 11 :77–97. doi: 10.1016/0198-8859(84)90047-8. [ PubMed ] [ CrossRef ] [ Google Scholar ]
- Denzin LK, Sant'Angelo DB, Hammond C, Surman MJ, Cresswell P. Negative regulation by HLA-DO of MHC class II-restricted antigen processing. Science. 1997; 278 :106–109. doi: 10.1126/science.278.5335.106. [ PubMed ] [ CrossRef ] [ Google Scholar ]
- Deverson EV, Gow IR, Coadwell WJ, Monaco JJ, Butcher GW, Howard JC. MHC class II region encoding proteins related to the multidrug resistance family of transmembrane transporters. Nature. 1990; 348 :738–741. doi: 10.1038/348738a0. [ PubMed ] [ CrossRef ] [ Google Scholar ]
- Garstka MA, Fritzsche S, Lenart I, Hein Z, Jankevicius G, Boyle LH, Elliott T, Trowsdale J, Antoniou AN, Zacharias M, Springer S. Tapasin dependence of major histocompatibility complex class I molecules correlates with their conformational flexibility. FASEB J. 2011; 25 :3989–3998. doi: 10.1096/fj.11-190249. [ PubMed ] [ CrossRef ] [ Google Scholar ]
- Glynne R, Powis SH, Beck S, Kelly A, Kerr LA, Trowsdale J. A proteasome-related gene between the two ABC transporter loci in the class II region of the human MHC. Nature. 1991; 353 :357–360. doi: 10.1038/353357a0. [ PubMed ] [ CrossRef ] [ Google Scholar ]
- Goodfellow PN, Jones EA, Van Heyningen V, Solomon E, Bobrow M, Miggiano V, Bodmer WF. The beta2-microglobulin gene is on chromosome 15 and not in the HL-A region. Nature. 1975; 254 :267–269. doi: 10.1038/254267a0. [ PubMed ] [ CrossRef ] [ Google Scholar ]
- Green DR, Flood PM, Gershon RK. Immunoregulatory T-cell pathways. Annu Rev Immunol. 1983; 1 :439–463. doi: 10.1146/annurev.iy.01.040183.002255. [ PubMed ] [ CrossRef ] [ Google Scholar ]
- Guce AI, Mortimer SE, Yoon T, Painter CA, Jiang W, Mellins ED, Stern LJ. HLA-DO acts as a substrate mimic to inhibit HLA-DM by a competitive mechanism. Nat Struct Mol Biol. 2013; 20 :90–98. doi: 10.1038/nsmb.2460. [ PMC free article ] [ PubMed ] [ CrossRef ] [ Google Scholar ]
- Hermann C, van Hateren A, Trautwein N, Neerincx A, Duriez PJ, Stevanovic S, Trowsdale J, Deane JE, Elliott T, Boyle LH (2015) TAPBPR alters MHC class I peptide presentation by functioning as a peptide exchange catalyst. Elife 4 [ PMC free article ] [ PubMed ]
- Horowitz A, Djaoud Z, Nemat-Gorgani N, Blokhuis J, Hilton HG, Beziat V, Malmberg KJ, Norman PJ, Guethlein LA, Parham P. Class I HLA haplotypes form two schools that educate NK cells in different ways. Sci Immunol. 2016; 1 :eaag1672. doi: 10.1126/sciimmunol.aag1672. [ PMC free article ] [ PubMed ] [ CrossRef ] [ Google Scholar ]
- Horton R, Gibson R, Coggill P, Miretti M, Allcock RJ, Almeida J, Forbes S, Gilbert JG, Halls K, Harrow JL, Hart E, Howe K, Jackson DK, Palmer S, Roberts AN, Sims S, Stewart CA, Traherne JA, Trevanion S, Wilming L, Rogers J, de Jong PJ, Elliott JF, Sawcer S, Todd JA, Trowsdale J, Beck S. Variation analysis and gene annotation of eight MHC haplotypes: the MHC haplotype project. Immunogenetics. 2008; 60 :1–18. doi: 10.1007/s00251-007-0262-2. [ PMC free article ] [ PubMed ] [ CrossRef ] [ Google Scholar ]
- Jiang J, Natarajan K, Boyd LF, Morozov GI, Mage MG, Margulies DH. Crystal structure of a TAPBPR-MHC I complex reveals the mechanism of peptide editing in antigen presentation. Science. 2017; 358 :1064–1068. doi: 10.1126/science.aao5154. [ PMC free article ] [ PubMed ] [ CrossRef ] [ Google Scholar ]
- Karre K, Ljunggren HG, Piontek G, Kiessling R. Selective rejection of H-2-deficient lymphoma variants suggests alternative immune defence strategy. Nature. 1986; 319 :675–678. doi: 10.1038/319675a0. [ PubMed ] [ CrossRef ] [ Google Scholar ]
- Kasahara M. The chromosomal duplication model of the major histocompatibility complex. Immunol Rev. 1999; 167 :17–32. doi: 10.1111/j.1600-065X.1999.tb01379.x. [ PubMed ] [ CrossRef ] [ Google Scholar ]
- Kaufman J. Co-evolution with chicken class I genes. Immunol Rev. 2015; 267 :56–71. doi: 10.1111/imr.12321. [ PubMed ] [ CrossRef ] [ Google Scholar ]
- Kaur G, Gras S, Mobbs JI, Vivian JP, Cortes A, Barber T, Kuttikkatte SB, Jensen LT, Attfield KE, Dendrou CA, Carrington M, McVean G, Purcell AW, Rossjohn J, Fugger L. Structural and regulatory diversity shape HLA-C protein expression levels. Nat Commun. 2017; 8 :15924. doi: 10.1038/ncomms15924. [ PMC free article ] [ PubMed ] [ CrossRef ] [ Google Scholar ]
- Kavathas P, Bach FH, DeMars R. Gamma ray-induced loss of expression of HLA and glyoxalase I alleles in lymphoblastoid cells. Proc Natl Acad Sci U S A. 1980; 77 :4251–4255. doi: 10.1073/pnas.77.7.4251. [ PMC free article ] [ PubMed ] [ CrossRef ] [ Google Scholar ]
- Kelly A, Powis SH, Glynne R, Radley E, Beck S, Trowsdale J. Second proteasome-related gene in the human MHC class II region. Nature. 1991; 353 :667–668. doi: 10.1038/353667a0. [ PubMed ] [ CrossRef ] [ Google Scholar ]
- Kelly A, Powis SH, Kerr LA, Mockridge I, Elliott T, Bastin J, Uchanska-Ziegler B, Ziegler A, Trowsdale J, Townsend A. Assembly and function of the two ABC transporter proteins encoded in the human major histocompatibility complex. Nature. 1992; 355 :641–644. doi: 10.1038/355641a0. [ PubMed ] [ CrossRef ] [ Google Scholar ]
- Kelly AP, Monaco JJ, Cho SG, Trowsdale J. A new human HLA class II-related locus, DM. Nature. 1991; 353 :571–573. doi: 10.1038/353571a0. [ PubMed ] [ CrossRef ] [ Google Scholar ]
- Klein J. Natural history of the major histocompatibility complex. New York: Wiley; 1986. [ Google Scholar ]
- Kobayashi KS, van den Elsen PJ. NLRC5: a key regulator of MHC class I-dependent immune responses. Nat Rev Immunol. 2012; 12 :813–820. doi: 10.1038/nri3339. [ PubMed ] [ CrossRef ] [ Google Scholar ]
- Kronenberg M, Steinmetz M, Kobori J, Kraig E, Kapp JA, Pierce CW, Sorensen CM, Suzuki G, Tada T, Hood L. RNA transcripts for I-J polypeptides are apparently not encoded between the I-A and I-E subregions of the murine major histocompatibility complex. Proc Natl Acad Sci U S A. 1983; 80 :5704–5708. doi: 10.1073/pnas.80.18.5704. [ PMC free article ] [ PubMed ] [ CrossRef ] [ Google Scholar ]
- Kulkarni S, Savan R, Qi Y, Gao X, Yuki Y, Bass SE, Martin MP, Hunt P, Deeks SG, Telenti A, Pereyra F, Goldstein D, Wolinsky S, Walker B, Young HA, Carrington M. Differential microRNA regulation of HLA-C expression and its association with HIV control. Nature. 2011; 472 :495–498. doi: 10.1038/nature09914. [ PMC free article ] [ PubMed ] [ CrossRef ] [ Google Scholar ]
- Larhammar D, Hammerling U, Rask L, Peterson PA. Sequence of gene and cDNA encoding murine major histocompatibility complex class II gene A beta 2. J Biol Chem. 1985; 260 :14111–14119. [ PubMed ] [ Google Scholar ]
- Li H, Ivarsson MA, Walker-Sperling VE, Subleski J, Johnson JK, Wright PW, Carrington M, Bjorkstrom NK, McVicar DW, Anderson SK. Identification of an elaborate NK-specific system regulating HLA-C expression. PLoS Genet. 2018; 14 :e1007163. doi: 10.1371/journal.pgen.1007163. [ PMC free article ] [ PubMed ] [ CrossRef ] [ Google Scholar ]
- Liljedahl M, Kuwana T, Fung-Leung WP, Jackson MR, Peterson PA, Karlsson L. HLA-DO is a lysosomal resident which requires association with HLA-DM for efficient intracellular transport. EMBO J. 1996; 15 :4817–4824. doi: 10.1002/j.1460-2075.1996.tb00862.x. [ PMC free article ] [ PubMed ] [ CrossRef ] [ Google Scholar ]
- Meissner TB, Li A, Biswas A, Lee KH, Liu YJ, Bayir E, Iliopoulos D, van den Elsen PJ, Kobayashi KS. NLR family member NLRC5 is a transcriptional regulator of MHC class I genes. Proc Natl Acad Sci U S A. 2010; 107 :13794–13799. doi: 10.1073/pnas.1008684107. [ PMC free article ] [ PubMed ] [ CrossRef ] [ Google Scholar ]
- Mellins E, Arp B, Ochs B, Erlich H, Pious D. A single amino acid substitution in the human histocompatibility leukocyte antigen DR3 beta chain selectively alters antigen presentation. J Exp Med. 1988; 168 :1531–1537. doi: 10.1084/jem.168.5.1531. [ PMC free article ] [ PubMed ] [ CrossRef ] [ Google Scholar ]
- Mellins E, Smith L, Arp B, Cotner T, Celis E, Pious D. Defective processing and presentation of exogenous antigens in mutants with normal HLA class II genes. Nature. 1990; 343 :71–74. doi: 10.1038/343071a0. [ PubMed ] [ CrossRef ] [ Google Scholar ]
- Monaco JJ, Cho S, Attaya M. Transport protein genes in the murine MHC: possible implications for antigen processing. Science. 1990; 250 :1723–1726. doi: 10.1126/science.2270487. [ PubMed ] [ CrossRef ] [ Google Scholar ]
- Monaco JJ, McDevitt HO. H-2-linked low-molecular weight polypeptide antigens assemble into an unusual macromolecular complex. Nature. 1984; 309 :797–799. doi: 10.1038/309797a0. [ PubMed ] [ CrossRef ] [ Google Scholar ]
- Morris P, Shaman J, Attaya M, Amaya M, Goodman S, Bergman C, Monaco JJ, Mellins E. An essential role for HLA-DM in antigen presentation by class II major histocompatibility molecules. Nature. 1994; 368 :551–554. doi: 10.1038/368551a0. [ PubMed ] [ CrossRef ] [ Google Scholar ]
- Neerincx A, Boyle LH. Properties of the tapasin homologue TAPBPR. Curr Opin Immunol. 2017; 46 :97–102. doi: 10.1016/j.coi.2017.04.008. [ PubMed ] [ CrossRef ] [ Google Scholar ]
- Neerincx A, Castro W, Guarda G, Kufer TA. NLRC5, at the heart of antigen presentation. Front Immunol. 2013; 4 :397. doi: 10.3389/fimmu.2013.00397. [ PMC free article ] [ PubMed ] [ CrossRef ] [ Google Scholar ]
- Neerincx A, Hermann C, Antrobus R, van Hateren A, Cao H, Trautwein N, Stevanovic S, Elliott T, Deane JE, Boyle LH (2017) TAPBPR bridges UDP-glucose:glycoprotein glucosyltransferase 1 onto MHC class I to provide quality control in the antigen presentation pathway. Elife 6 [ PMC free article ] [ PubMed ]
- Norman PJ, Hollenbach JA, Nemat-Gorgani N, Marin WM, Norberg SJ, Ashouri E, Jayaraman J, Wroblewski EE, Trowsdale J, Rajalingam R, Oksenberg JR, Chiaroni J, Guethlein LA, Traherne JA, Ronaghi M, Parham P. Defining KIR and HLA class I genotypes at highest resolution via high-throughput sequencing. Am J Hum Genet. 2016; 99 :375–391. doi: 10.1016/j.ajhg.2016.06.023. [ PMC free article ] [ PubMed ] [ CrossRef ] [ Google Scholar ]
- Ortmann B, Copeman J, Lehner PJ, Sadasivan B, Herberg JA, Grandea AG, Riddell SR, Tampe R, Spies T, Trowsdale J, Cresswell P. A critical role for tapasin in the assembly and function of multimeric MHC class I-TAP complexes. Science. 1997; 277 :1306–1309. doi: 10.1126/science.277.5330.1306. [ PubMed ] [ CrossRef ] [ Google Scholar ]
- Park B, Lee S, Kim E, Ahn K. A single polymorphic residue within the peptide-binding cleft of MHC class I molecules determines spectrum of tapasin dependence. J Immunol. 2003; 170 :961–968. doi: 10.4049/jimmunol.170.2.961. [ PubMed ] [ CrossRef ] [ Google Scholar ]
- Paul P, van den Hoorn T, Jongsma ML, Bakker MJ, Hengeveld R, Janssen L, Cresswell P, Egan DA, van Ham M, Ten Brinke A, Ovaa H, Beijersbergen RL, Kuijl C, Neefjes J. A genome-wide multidimensional RNAi screen reveals pathways controlling MHC class II antigen presentation. Cell. 2011; 145 :268–283. doi: 10.1016/j.cell.2011.03.023. [ PubMed ] [ CrossRef ] [ Google Scholar ]
- Petersdorf EW, Gooley TA, Malkki M, Bacigalupo AP, Cesbron A, Du Toit E, Ehninger G, Egeland T, Fischer GF, Gervais T, Haagenson MD, Horowitz MM, Hsu K, Jindra P, Madrigal A, Oudshoorn M, Ringden O, Schroeder ML, Spellman SR, Tiercy JM, Velardi A, Witt CS, O'Huigin C, Apps R, Carrington M, International Histocompatibility Working Group in Hematopoietic Cell T HLA-C expression levels define permissible mismatches in hematopoietic cell transplantation. Blood. 2014; 124 :3996–4003. doi: 10.1182/blood-2014-09-599969. [ PMC free article ] [ PubMed ] [ CrossRef ] [ Google Scholar ]
- Pious D, Dixon L, Levine F, Cotner T, Johnson R. HLA class II regulation and structure. Analysis with HLA-DR3 and HLA-DP point mutants. J Exp Med. 1985; 162 :1193–1207. doi: 10.1084/jem.162.4.1193. [ PMC free article ] [ PubMed ] [ CrossRef ] [ Google Scholar ]
- Porter KM, Hermann C, Traherne JA, Boyle LH. TAPBPR isoforms exhibit altered association with MHC class I. Immunology. 2014; 142 :289–299. doi: 10.1111/imm.12253. [ PMC free article ] [ PubMed ] [ CrossRef ] [ Google Scholar ]
- Powis SJ, Deverson EV, Coadwell WJ, Ciruela A, Huskisson NS, Smith H, Butcher GW, Howard JC. Effect of polymorphism of an MHC-linked transporter on the peptides assembled in a class I molecule. Nature. 1992; 357 :211–215. doi: 10.1038/357211a0. [ PubMed ] [ CrossRef ] [ Google Scholar ]
- Powis SJ, Young LL, Joly E, Barker PJ, Richardson L, Brandt RP, Melief CJ, Howard JC, Butcher GW. The rat cim effect: TAP allele-dependent changes in a class I MHC anchor motif and evidence against C-terminal trimming of peptides in the ER. Immunity. 1996; 4 :159–165. doi: 10.1016/S1074-7613(00)80680-9. [ PubMed ] [ CrossRef ] [ Google Scholar ]
- Ramsuran V, Naranbhai V, Horowitz A, Qi Y, Martin MP, Yuki Y, Gao X, Walker-Sperling V, Del Prete GQ, Schneider DK, Lifson JD, Fellay J, Deeks SG, Martin JN, Goedert JJ, Wolinsky SM, Michael NL, Kirk GD, Buchbinder S, Haas D, Ndung'u T, Goulder P, Parham P, Walker BD, Carlson JM, Carrington M. Elevated HLA-A expression impairs HIV control through inhibition of NKG2A-expressing cells. Science. 2018; 359 :86–90. doi: 10.1126/science.aam8825. [ PMC free article ] [ PubMed ] [ CrossRef ] [ Google Scholar ]
- Reeves E, Colebatch-Bourn A, Elliott T, Edwards CJ, James E. Functionally distinct ERAP1 allotype combinations distinguish individuals with ankylosing spondylitis. Proc Natl Acad Sci U S A. 2014; 111 :17594–17599. doi: 10.1073/pnas.1408882111. [ PMC free article ] [ PubMed ] [ CrossRef ] [ Google Scholar ]
- Reeves E, Colebatch-Bourn A, Elliott T, Edwards CJ, James E. Reply to Robinson and Brown: it is the combination of ERAP1 allotypes that identifies individuals with ankylosing spondylitis. Proc Natl Acad Sci U S A. 2015; 112 :E1817. doi: 10.1073/pnas.1502270112. [ PMC free article ] [ PubMed ] [ CrossRef ] [ Google Scholar ]
- Reeves E, Elliott T, James E, Edwards CJ. ERAP1 in the pathogenesis of ankylosing spondylitis. Immunol Res. 2014; 60 :257–269. doi: 10.1007/s12026-014-8576-2. [ PubMed ] [ CrossRef ] [ Google Scholar ]
- Reith W, Mach B. The bare lymphocyte syndrome and the regulation of MHC expression. Annu Rev Immunol. 2001; 19 :331–373. doi: 10.1146/annurev.immunol.19.1.331. [ PubMed ] [ CrossRef ] [ Google Scholar ]
- Robinson PC, Brown MA. ERAP1 biology and assessment in ankylosing spondylitis. Proc Natl Acad Sci U S A. 2015; 112 :E1816. doi: 10.1073/pnas.1501475112. [ PMC free article ] [ PubMed ] [ CrossRef ] [ Google Scholar ]
- Sakaguchi S, Wing K, Miyara M. Regulatory T cells—a brief history and perspective. Eur J Immunol. 2007; 37 (Suppl 1):S116–S123. doi: 10.1002/eji.200737593. [ PubMed ] [ CrossRef ] [ Google Scholar ]
- Salter RD, Howell DN, Cresswell P. Genes regulating HLA class I antigen expression in T-B lymphoblast hybrids. Immunogenetics. 1985; 21 :235–246. doi: 10.1007/BF00375376. [ PubMed ] [ CrossRef ] [ Google Scholar ]
- Shiina T, Inoko H, Kulski JK. An update of the HLA genomic region, locus information and disease associations: 2004. Tissue Antigens. 2004; 64 :631–649. doi: 10.1111/j.1399-0039.2004.00327.x. [ PubMed ] [ CrossRef ] [ Google Scholar ]
- Spies T, Bresnahan M, Bahram S, Arnold D, Blanck G, Mellins E, Pious D, DeMars R. A gene in the human major histocompatibility complex class II region controlling the class I antigen presentation pathway. Nature. 1990; 348 :744–747. doi: 10.1038/348744a0. [ PubMed ] [ CrossRef ] [ Google Scholar ]
- Spies T, DeMars R. Restored expression of major histocompatibility class I molecules by gene transfer of a putative peptide transporter. Nature. 1991; 351 :323–324. doi: 10.1038/351323a0. [ PubMed ] [ CrossRef ] [ Google Scholar ]
- Spring B, Fonatsch C, Muller C, Pawelec G, Kompf J, Wernet P, Ziegler A. Refinement of HLA gene mapping with induced B-cell line mutants. Immunogenetics. 1985; 21 :277–291. doi: 10.1007/BF00375380. [ PubMed ] [ CrossRef ] [ Google Scholar ]
- Staehli F, Ludigs K, Heinz LX, Seguin-Estevez Q, Ferrero I, Braun M, Schroder K, Rebsamen M, Tardivel A, Mattmann C, MacDonald HR, Romero P, Reith W, Guarda G, Tschopp J. NLRC5 deficiency selectively impairs MHC class I-dependent lymphocyte killing by cytotoxic T cells. J Immunol. 2012; 188 :3820–3828. doi: 10.4049/jimmunol.1102671. [ PubMed ] [ CrossRef ] [ Google Scholar ]
- Steimle V, Otten LA, Zufferey M, Mach B. Complementation cloning of an MHC class II transactivator mutated in hereditary MHC class II deficiency (or bare lymphocyte syndrome) Cell. 1993; 75 :135–146. doi: 10.1016/S0092-8674(05)80090-X. [ PubMed ] [ CrossRef ] [ Google Scholar ]
- Steinmetz M, Hood L. Genes of the major histocompatibility complex in mouse and man. Science. 1983; 222 :727–733. doi: 10.1126/science.6356354. [ PubMed ] [ CrossRef ] [ Google Scholar ]
- Teng MS, Stephens R, Du Pasquier L, Freeman T, Lindquist JA, Trowsdale J. A human TAPBP (TAPASIN)-related gene, TAPBP-R. Eur J Immunol. 2002; 32 :1059–1068. doi: 10.1002/1521-4141(200204)32:4<1059::AID-IMMU1059>3.0.CO;2-G. [ PubMed ] [ CrossRef ] [ Google Scholar ]
- Timms RT, Menzies SA, Tchasovnikarova IA, Christensen LC, Williamson JC, Antrobus R, Dougan G, Ellgaard L, Lehner PJ. Genetic dissection of mammalian ERAD through comparative haploid and CRISPR forward genetic screens. Nat Commun. 2016; 7 :11786. doi: 10.1038/ncomms11786. [ PMC free article ] [ PubMed ] [ CrossRef ] [ Google Scholar ]
- Tonnelle C, DeMars R, Long EO. DO beta: a new beta chain gene in HLA-D with a distinct regulation of expression. EMBO J. 1985; 4 :2839–2847. doi: 10.1002/j.1460-2075.1985.tb04012.x. [ PMC free article ] [ PubMed ] [ CrossRef ] [ Google Scholar ]
- Townsend A, Ohlen C, Bastin J, Ljunggren HG, Foster L, Karre K. Association of class I major histocompatibility heavy and light chains induced by viral peptides. Nature. 1989; 340 :443–448. doi: 10.1038/340443a0. [ PubMed ] [ CrossRef ] [ Google Scholar ]
- Townsend AR, Gotch FM, Davey J. Cytotoxic T cells recognize fragments of the influenza nucleoprotein. Cell. 1985; 42 :457–467. doi: 10.1016/0092-8674(85)90103-5. [ PubMed ] [ CrossRef ] [ Google Scholar ]
- Tregaskes CA, Harrison M, Sowa AK, van Hateren A, Hunt LG, Vainio O, Kaufman J. Surface expression, peptide repertoire, and thermostability of chicken class I molecules correlate with peptide transporter specificity. Proc Natl Acad Sci U S A. 2016; 113 :692–697. doi: 10.1073/pnas.1511859113. [ PMC free article ] [ PubMed ] [ CrossRef ] [ Google Scholar ]
- Trowsdale J, Hanson I, Mockridge I, Beck S, Townsend A, Kelly A. Sequences encoded in the class II region of the MHC related to the ‘ABC’ superfamily of transporters. Nature. 1990; 348 :741–744. doi: 10.1038/348741a0. [ PubMed ] [ CrossRef ] [ Google Scholar ]
- Trowsdale J, Kelly A. The human HLA class II alpha chain gene DZ alpha is distinct from genes in the DP, DQ and DR subregions. EMBO J. 1985; 4 :2231–2237. doi: 10.1002/j.1460-2075.1985.tb03919.x. [ PMC free article ] [ PubMed ] [ CrossRef ] [ Google Scholar ]
- Turner TR, Hayhurst JD, Hayward DR, Bultitude WP, Barker DJ, Robinson J, Madrigal JA, Mayor NP, Marsh SGE. Single molecule real-time DNA sequencing of HLA genes at ultra-high resolution from 126 international HLA and immunogenetics workshop cell lines. HLA. 2018; 91 :88–101. doi: 10.1111/tan.13184. [ PubMed ] [ CrossRef ] [ Google Scholar ]
- van Ham SM, Tjin EP, Lillemeier BF, Gruneberg U, van Meijgaarden KE, Pastoors L, Verwoerd D, Tulp A, Canas B, Rahman D, Ottenhoff TH, Pappin DJ, Trowsdale J, Neefjes J. HLA-DO is a negative modulator of HLA-DM-mediated MHC class II peptide loading. Curr Biol. 1997; 7 :950–957. doi: 10.1016/S0960-9822(06)00414-3. [ PubMed ] [ CrossRef ] [ Google Scholar ]
- Williams AP, Peh CA, Purcell AW, McCluskey J, Elliott T. Optimization of the MHC class I peptide cargo is dependent on tapasin. Immunity. 2002; 16 :509–520. doi: 10.1016/S1074-7613(02)00304-7. [ PubMed ] [ CrossRef ] [ Google Scholar ]
- Zimmer J, Andres E, Donato L, Hanau D, Hentges F, de la Salle H. Clinical and immunological aspects of HLA class I deficiency. QJM. 2005; 98 :719–727. doi: 10.1093/qjmed/hci112. [ PubMed ] [ CrossRef ] [ Google Scholar ]
- Zinkernagel RM, Doherty PC. Immunological surveillance against altered self components by sensitised T lymphocytes in lymphocytic choriomeningitis. Nature. 1974; 251 :547–548. doi: 10.1038/251547a0. [ PubMed ] [ CrossRef ] [ Google Scholar ]
Antigen presentation in cancer: insights into tumour immunogenicity and immune evasion
Affiliations.
- 1 Genentech Inc., South San Francisco, CA, USA. [email protected].
- 2 Genentech Inc., South San Francisco, CA, USA.
- 3 Genentech Inc., South San Francisco, CA, USA. [email protected].
- PMID: 33750922
- DOI: 10.1038/s41568-021-00339-z
Immune checkpoint blockade, which blocks inhibitory signals of T cell activation, has shown tremendous success in treating cancer, although success still remains limited to a fraction of patients. To date, clinically effective CD8 + T cell responses appear to target predominantly antigens derived from tumour-specific mutations that accumulate in cancer, also called neoantigens. Tumour antigens are displayed on the surface of cells by class I human leukocyte antigens (HLA-I). To elicit an effective antitumour response, antigen presentation has to be successful at two distinct events: first, cancer antigens have to be taken up by dendritic cells (DCs) and cross-presented for CD8 + T cell priming. Second, the antigens have to be directly presented by the tumour for recognition by primed CD8 + T cells and killing. Tumours exploit multiple escape mechanisms to evade immune recognition at both of these steps. Here, we review the tumour-derived factors modulating DC function, and we summarize evidence of immune evasion by means of quantitative modulation or qualitative alteration of the antigen repertoire presented on tumours. These mechanisms include modulation of antigen expression, HLA-I surface levels, alterations in the antigen processing and presentation machinery in tumour cells. Lastly, as complete abrogation of antigen presentation can lead to natural killer (NK) cell-mediated tumour killing, we also discuss how tumours can harbour antigen presentation defects and still evade NK cell recognition.
Publication types
- Antigen Presentation / immunology*
- Antigens, Neoplasm / immunology*
- CD8-Positive T-Lymphocytes / immunology*
- Immune Evasion / immunology*
- Immunomodulation
- Lymphocytes, Tumor-Infiltrating / immunology*
- Neoplasms / immunology*
- Neoplasms / pathology
- Antigens, Neoplasm
Advertisement
Genetics of antigen processing and presentation
- Open access
- Published: 13 September 2018
- Volume 71 , pages 161–170, ( 2019 )
Cite this article
You have full access to this open access article
- Adrian Kelly 1 &
- John Trowsdale 1
8743 Accesses
68 Citations
5 Altmetric
Explore all metrics
Immune response to disease requires coordinated expression of an army of molecules. The highly polymorphic MHC class I and class II molecules are key to control of specificity of antigen presentation. Processing of the antigen, to peptides or other moieties, requires other sets of molecules. For classical class I, this includes TAP peptide transporters, proteasome components and Tapasin, genes which are encoded within the MHC. Similarly, HLA-DO and -DM, which influence presentation by HLA class II molecules, are encoded in the MHC region. Analysis of MHC mutants, including point mutations and large deletions, has been central to understanding the roles of these genes. Mouse genetics has also played a major role. Many other genes have been identified including those controlling expression of HLA class I and class II at the transcriptional level. Another genetic approach that has provided insight has been the analysis of microorganisms, including viruses and bacteria that escape immune recognition by blocking these antigen processing and presentation pathways. Here, we provide a brief history of the genetic approaches, both traditional and modern, that have been used in the quest to understand antigen processing and presentation.
Similar content being viewed by others
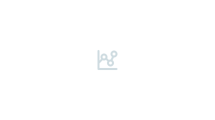
Introduction: MHC/KIR and governance of specificity
Evolving insights for mhc class ii antigen processing and presentation in health and disease.
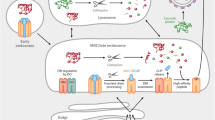
A personal retrospective on the mechanisms of antigen processing
Avoid common mistakes on your manuscript.
Some history
The early history of the genetics of antigen processing and presentation followed from the work on histocompatibility in mice, initially by Peter Gorer, who worked at the Lister Institute and Guy’s hospital in London. His discovery of the MHC in turn was inspired by three developments. First was the curious pastime of inbreeding mouse strains, a fashionable hobby which spread from China. It reached America in the early 1900s, and after a while, pioneering geneticists realised the advantage of inbred strains for research. Many of the mouse strains were started over 100 years ago. C57BL, one of the original strains, was designated as the mice were black and were number 57. BALB/c mice were white, on the other hand, and were designated by their originator, Halsey J. Bagg, as Bagg albino, or BALB/c for short. A fascinating early history of these developments is presented in a book on the natural history of the MHC (Klein 1986 ).
Inbred strains were pivotal in the next development, where researchers used them to study the genetics of tumour rejection. As early as 1903, it was discovered that tumours that grew well when transferred within the same strain were rejected in a different one. Then, in 1922, Little and Johnson showed that transplantation of normal tissue was subject to the same strain specificity as tumours. A third stimulus was the development of blood group research, largely attributed to Landsteiner.
JB Haldane suggested that tumour resistance factors may be akin to blood group antigens, but it was Gorer who performed experiments to test the idea that antigens were shared by both malignant and normal tissues. This led to the formulation of an immunological theory of transplantation, which was later systematised by Peter Medawar. George Snell was studying similar phenomena, and after collaborating with Gorer, he proposed calling the tumour-resistance factors Histocompatibility genes. His approach started to reveal some of the complexity of histocompatibility.
Early work leading to the discovery of the human HLA complex developed in the 1950s and was dependent on the study of antibodies against alloantigens on white blood cells by three laboratories: Jean Dausset in Paris, Rose Payne and Walter Bodmer in Stanford, and Jon van Rood in Leiden. It was realised that some patients, and women who had borne several children, tended to make such antibodies, which were independent of ABO blood groups and erythrocytes. At the time, human organ transplantation was becoming widespread, and it gradually became accepted that human leukocyte antigens were the equivalent of mouse H-2 antigens. The hope was that careful matching, as in ABO, could lead to organ transplants that were not rejected. It was soon realised that the HLA system was more complex than ABO and progress depended on exchange of cells and antisera. The International Histocompatibility Workshops , which have been held every few years since 1964, were critical in interpreting and integrating information obtained with a variety of techniques from different laboratories. Analysis of data from these workshops indicated that a single genetic region was pivotal, namely, in humans, HLA, the Major Histocompatibility Complex. An associated protein chain, β2microglobulin, was identified as a component of HLA antigens and was later mapped outside the complex to chromosome 15 (Goodfellow et al. 1975 ).
Attempts to develop in vitro assays to study graft rejection led to the mixed lymphocyte reaction (MLR), which also turned out to be controlled by the MHC region. However, the results did not correlate completely with the serologically defined determinants. Work in both human and mouse indicated that these determinants, as well as MLR responsiveness, were both part of the H2 and HLA complexes but were separated genetically. Meanwhile, a different set of experiments showed that levels of antibody response to short synthetic polypeptides were controlled by the MHC. For example, C57 mice responded well to the branched synthetic polypeptide (T, G)-A-L, but CBA animals were poor responders. These effects were traced to the H-2 region leading to the so-called immune response (Ir) genes (Benacerraf and McDevitt 1972 ).
These many years of work, all indicated that the MHC was a major hub controlling a number of immunological phenomena. Indeed, additional experiments showed that the MHC also controlled susceptibility to viruses. Work in Canberra showed that cytotoxic T cells simultaneously recognised viral antigens and MHC molecules, in a phenomenon that came to be known as MHC restriction (Zinkernagel and Doherty 1974 ).
The nature of the MHC became even more complex when it was proposed that T cell suppression was also controlled by the class II region (Green et al. 1983 ). There followed a decade of controversy over the existence of this phenomenon (Bloom et al. 1992 ). It was eventually accepted, and the responsible T cells were called regulatory to distinguish them from the confusing history of suppression (Sakaguchi et al. 2007 ). The genetics behind the controversy concerned the I-J gene which was supposed to map to the I region of the mouse MHC and control the function of suppressor T cells. The I-J antigens were proposed to be soluble molecules secreted by suppressor T cells. It was a shock to find that discrete I-J genes did not exist once the I region of the mouse had been cloned (Kronenberg et al. 1983 ).
The genetics of antigen processing and presentation was advanced dramatically in the late 1980s when two further major technical developments helped to get to grips with the complexity. One was the DNA cloning revolution and the second was the determination of the structure of MHC molecules from crystals. Initial cloning of H-2 and HLA antigen genes led quickly to assembly of maps of the MHC in humans and mice. These dramatically simplified the picture to just a handful of class I and class II loci, albeit with a profound level of polymorphism. The early maps of mouse MHC were painstakingly assembled from overlapping cosmids (Steinmetz and Hood 1983 ). Many different human haplotypes have been analysed by these techniques (Horton et al. 2008 ; Shiina et al. 2004 ), as well as using more modern, high-throughput approaches (Norman et al. 2016 ).
The nature of the polymorphism was mysterious, as class I and class II chains encompassed many amino acid changes seemingly scattered throughout the first two domains of class I and the first domains of both chains of class II. The breakthrough came with the crystal structure of the first MHC antigen, HLA-A2 by Pamela Bjorkman, who was a PhD student at the time in the Wiley/Strominger laboratories (Bjorkman et al. 1987a , Bjorkman et al. 1987b ). The realisation that class I and class II molecules possessed a groove which bound peptides immediately swept away other models of antigen recognition, such as those invoking independent receptors for antigen and histocompatibility molecules on T cells.
The development of molecular immunology through the creation of mutants, DNA sequencing, protein structure and gene discovery then paved the way for uncovering the various components of the antigen processing and presentation pathways, as outlined below (Table 1 ).
Mutant cell lines
Panels of HLA homozygous typing cell lines have been important tools in the analysis of human tissue types through HLA classes I and II. These lines were derived by Epstein Barr Virus transformation of lymphoid cells from consanguineous mating, usually first cousin. They have been used for over 40 years by the HLA community and are still in use today (Turner et al. 2018 ).
Analysis of the involvement of the HLA region in antigen processing and presentation was further enhanced by the generation of deletions and other mutations in lymphoblastoid cell lines (Fig. 1 ). One series of mutants was made by γ ray mutagenesis of the cell line B-LCL721 by the DeMars group (DeMars et al. 1984 ; Kavathas et al. 1980 ). Cells were subjected to 2 cycles of mutagenesis followed by immune-selection with monoclonal antibodies for MHC antigen loss. B-LCL721 contains the following two MHC haplotypes:
HLA-A*01 HLA-B*08 HLA-DRB1*03 HLA-DPB1*04
HLA-A*02 HLA-B*51 HLA-DRB1*01 HLA-DPB1*02
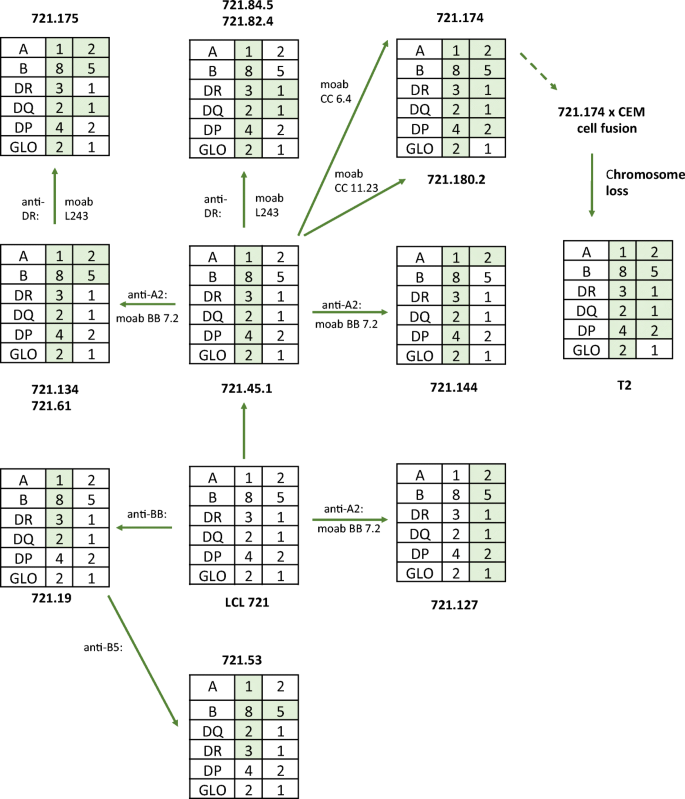
Composition of the mutant lines derived in the DeMars laboratory (DeMars et al. 1984 ; Kavathas et al. 1980 ) and the further derivative T2 (Salter et al. 1985 ). The shaded boxes show the proposed extents of the deletions. See text for more details of typing. These mutant cells have been used in numerous antigen processing and presentation studies. The Figure adapted from Demars et al. 1984
More details on the class I HLA types are available on the IPD-IMGT/HLA database. Footnote 1 To our knowledge, not all class II genes have been typed in these cells. Mutant cell lines were isolated by subjecting these cells to irradiation. After 5 days growth, the survivors were selected with complement and a variety of monoclonal antibodies. Analysis of these mutants showed that they contained large homozygous or hemizygous deletions over the MHC region (Fig. 1 ). One such line LCL721.174, and its derivative 174xCEM.T2, has been used extensively to investigate TAP transporter function and MHC class I peptide loading. 174xCEM.T2, more commonly referred to as T2, was generated by fusing LCL721.174 with CEMR.3, a T-LCL, and selecting for loss of CEMR.3-derived copies of chromosome 6 (Salter et al. 1985 ). Both 174XCEM.T2 and LCL721.174 therefore carry the same chromosome 6 MHC deletion.
A similar procedure was used by Andreas Ziegler, using the lymphoma cell line BJAB.B95.8.6 (Spring et al. 1985 ). The wild-type cells were typed with the resolution available at that time as:
HLA-A*1 HLA-B*35 HLA-C*4 HLA-DR*5 HLA-DQw3 HLA-DP*4
HLA-A*2 HLA-B*13 HLA-C* HLA-DR* HLA-DQw1 HLA-DP*2
The Pious laboratory used a variation of this approach to isolate mutants in class II (8.1.6 and 9.28.6) by mutagenizing with EMS an HLA-DR3+ cell line, T5-1 (Mellins et al. 1988 ; Pious et al. 1985 ). Similarly, in mouse studies designed to investigate the nature of self and non-self-recognition, Klaus Karre generated a murine cell line RMA-S that lacked surface H-2 expression but was rejected in tumour transplant models (Karre et al. 1986 ). Analysis of this cell line was instrumental in providing early models for peptide loading by MHC class I molecules (Townsend et al. 1989 ). In addition, Roberto Accolla irradiated Raji cells to produce a mutant line RJ2.2.5 selecting against HLA-DR expression with an antibody D1–12(Accolla 1983 ), facilitating identification of CIITA.
These mutant cell lines and their derivatives have been instrumental in a long series of experimental approaches to uncover various components of antigen processing, and they continue to be important tools in investigating their mechanism of action.
TAP transporters
A key insight into antigen processing was the finding that influenza A-specific cytotoxic T lymphocytes (CTL) recognise discrete epitopes of the nucleoprotein molecule. These data implied a mechanism for generation of viral protein fragments and their transport to the cell surface for presentation to CTL (Townsend et al. 1985 ). The peptide-binding groove in HLA molecules was a strong contender for the peptide carrier. There was a problem though in that MHC molecules were integrated into the cell membrane, so how did peptide fragments, which lacked signal sequences, access the endoplasmic reticulum (ER) to be loaded? How were the peptides transported to the cell surface on MHC molecules?
The solution came from MHC mapping and analysis of the mutants referred to above. Several groups simultaneously identified genes encoding transporter molecules mapping to the MHC. These were transporters of the so-called ABC (ATP-binding cassette) type, which were subsequently named TAP, for Transporters Associated with Antigen Processing (Deverson et al. 1990 ; Monaco et al. 1990 ; Spies et al. 1990 ; Trowsdale et al. 1990 ). These data suggested immediately that the transporters, encoded as a heterodimer of two proteins, TAP1 and TAP2, were responsible for pumping peptides into the ER (Kelly et al. 1992 ). To confirm this, the TAP1 sequence restored HLA class I expression when transfected into a cell line with a mutant TAP1 gene, LCL721.134 (Spies and DeMars 1991 ), and the TAP2 sequence restored function and co-precipitation of TAP1 and TAP2 when transfected into cells deficient in TAP2 (Kelly et al. 1992 ). However, this was not the case when using LCL721.174 cells, which maintained a large deletion over the class II region, covering both components of the heterodimer, TAP1 and TAP2(Spies and DeMars 1991 ). Studies of rats and chickens were particularly informative as the TAP genes are polymorphic in these species. It turned out that specific allelic versions of TAP supply peptides appropriate for class I molecules whose genes are linked in cis on the haplotype. Inappropriate pairing of TAP alleles with class I alleles they serve with non-binding peptides led to reduced expression of class I, which could be detected at the cell surface (Deverson et al. 1990 ; Kaufman 2015 ; Powis et al. 1996 ).
Natural mutants in TAP
A number of individuals have been identified with immune-deficiencies due to mutations in TAP (de la Salle et al. 1994 ; Zimmer et al. 2005 ). These belong to the category of type I bare lymphocyte (BLS) syndrome, where HLA class I, but not class II, is affected. These patients generally have a severe reduction of class I at the cell surface, but in spite of this, most of these individuals reach adulthood. They are rare and are almost exclusively HLA homozygous due to first cousin parentage. They generally display chronic infections of the respiratory tract (purulent rhinitis, pansinusitis, otitis media) with bacteria ( H. influenzae , S. pneumoniae , S. aureus , K. pneumoniae , E. coli and P. aeruginosa ). About half of them exhibit granulomatous skin lesions. This may be due to suboptimal cytokine and cellular responses, leading to tissue damage and favouring subsequent bacterial infection and further recruitment of phagocytic cells. Indeed, antibiotics that impair neutrophils appear to be beneficial.
Patients with defects in TAP do not suffer from severe viral infection. This is unexpected but may relate to the fact that they have a normal humoral (antibody) response and significant numbers of T cells, particularly γδT cells, as well as NK cells and neutrophils. Additionally, presentation of some viral antigens is TAP-independent. Another symptom in these patients is necrotizing granulomatous lesions particularly around the nose, resulting in loss of the septum and associated cartilage. Presentation is variable though. The skin ulcers were found to contain macrophages and NK cells.
In terms of biological effects, the proportion of T cells may be reduced in patients with TAP defects, but there is wide variation in phenotype. They tend to have a higher proportion of γδT cells. Interestingly, numbers of NK cells are normal, but they have no cytotoxic activity against the standard class I-negative targets, unless activated by cytokines.
Proteasome components—LMPs
At the same time that TAPs were discovered, clues to the proteolytic machinery, responsible for breaking the antigenic proteins into peptides, were also provided, by studying mutants over the MHC region. The biochemical prelude to this discovery came in the early 1980s when Monaco and McDevitt described a series of up to16 low-molecular weight proteins , LMPs, that mapped to the MHC. Initially, the functions of these proteins were not obvious, but they formed a complex by co-precipitation and varied between different mouse haplotypes, in other words were polymorphic (Monaco and McDevitt 1984 ). It was subsequently established that genes for two interferon-inducible, catalytic proteasome subunits, LMP2 (PSMB9; β1i) and LMP7 (PSMB8: β5i), were closely linked to the TAP1 and TAP2 genes in both human and mouse MHC (Glynne et al. 1991 ; Kelly et al. 1991a ). As with the TAPs, finding proteasome components encoded in the MHC immediately suggested that the proteasome was responsible for producing the antigenic peptides. Proteasomes are ubiquitous cellular components responsible for continual turnover of proteins in general. However, the MHC-encoded proteasome genes were interferon inducible and, when expressed, replaced constitutive components in a subset of the main structures. Another interferon-inducible subunit PSMB10 (β2i) is encoded outside the MHC on chromosome 16q22.1.
ERp57 and ERAP1
A number of chaperones are associated with class I as it matures and picks up peptide in the peptide-loading complex. These chaperones serve multiple proteins in the ER and are not dedicated to class I. However, ERp57 is a component of the peptide-loading complex. The protein aids folding of nascent glycoproteins by facilitating disulphide bond isomerisation.
ERAP1 is an amino peptidase. It plays a role in determining the length and sequence of peptides bound and presented by class I allotypes. HLA-B27 is strongly associated with ankylosing spondylitis (Chen et al. 2014 ), and genetic studies indicated that certain ERAP1 allotypes are more associated with ankylosing spondylitis than others, due to their contribution to peptide trimming (Reeves et al. 2014a , Reeves et al. 2014b ). There has been some controversy over this effect as it was difficult to analyse genetically. It was proposed to involve specific combinations of different ERAP1 allotypes, making association studies difficult (Reeves et al. 2015 ; Robinson and Brown 2015 ).
The gene for Tapasin, another component of the antigen processing machinery, is located just outside the MHC region (Ortmann et al. 1997 ). The product of this gene, which is an additional member of the immunoglobulin gene family, links the peptide transporter to the nascent class I molecule in a complex, the peptide-loading complex (Blees et al. 2017 ). Optimisation of the MHC class I peptide cargo is proposed to be dependent on Tapasin (Williams et al. 2002 ). However, class I molecules vary in their dependence on the protein, an effect that has been mapped to specific positions on the HLA molecule (Park et al. 2003 ) as well as to its conformational flexibility (Garstka et al. 2011 ). For example, the HLA-B*44 allele, which differs exclusively at position 156, B*44:02 (156Asp), is Tapasin-dependent, whereas other alleles are not (Badrinath et al. 2012 ). The reason for this is not known but could relate to the fact that some virus products compromise antigen presentation by interacting with the Tapasin protein.
There is evidence that the jawed vertebrate genome has undergone duplication at least twice in its evolutionary history, according to the 2R hypothesis. Accordingly, it was demonstrated that there are traces of at least four clusters of genes related to those in the MHC proper on chromosome 6 (Kasahara 1999 ). One paralogous region, identified by Louis Du Pasquier, was at chromosome position 12p13.3. A gene in this region shared sequence homology with Tapasin, dubbed TAPBPR (Teng et al. 2002 ). The product of this gene was subsequently shown to be a novel component of the MHC class I presentation pathway, which acts as a peptide exchange catalyst (Hermann et al. 2015 ; Neerincx and Boyle 2017 ). TAPBPR interacts with class I in a similar manner to Tapasin but is not complexed to TAP (Jiang et al. 2017 ). TAPBPR links UDP-glucose:glycoprotein glucosyltransferase 1 onto MHC class I (Neerincx et al. 2017 ). Tapasin is monomorphic, but TAPBPR is relatively polymorphic and there are a number of common alleles and splice variants in humans (Porter et al. 2014 ). The relationship between the products of these alleles and MHC Class I loci and alleles is not known. As with Tapasin, it appears that some alleles of class I are dependent, and others independent, of the molecule.
HLA-DM and DO
The existence of an MHC-encoded factor that was essential for MHC class II antigen presentation was first suggested through the characterisation of mutagenised B lymphoblastoid cells selected for loss of HLA-DR3 reactivity with a DR3-specific antibody, 16:23(Mellins et al. 1990 ). These cells had normal levels of cell surface DR but were unable to present native antigen to T cells. They possessed class II molecules that were predominantly filled with the CLIP peptide and fell apart in the presence of SDS. The defect was eventually mapped to HLA-DM (Morris et al. 1994 ), a class II-related molecule previously identified in both mouse and human in screens for expressed genes encoded on cosmids spanning the MHC region (Kelly et al. 1991b ; Cho et al. 1991 ). HLA-DM acts as a peptide editing chaperone stabilising class II whilst exchanging CLIP for antigenic peptide. DM activity is regulated by a second non-classical class II molecule, now called HLA-DO. HLA-DO alpha and beta chains were first identified due to their high homology with human and murine classical class II molecules (Larhammar et al. 1985 ; Tonnelle et al. 1985 ; Trowsdale and Kelly 1985 ). It proved much harder to decipher the role of DO even though the molecule was identified some 6 years before DM. DOA and DOB were not located together in the MHC, and the chains showed different mRNA expression patterns initially suggesting that they would not associate as a pair. DO resides in late endosomal compartments but requires association with DM in the endoplasmic reticulum for correct assembly (Liljedahl et al. 1996 ). The in vivo role of DO is still debatable, but mechanistically, it functions as a negative regulator of DM activity (van Ham et al. 1997 ; Denzin et al. 1997 ). Unlike the transient interactions seen between DM and DR, the DM/DO interaction is very stable, suggesting that DO acts as a competitive inhibitor of DM, a view consistent with the DM/DO crystal structure (Guce et al. 2013 ).
Haplotypes and linkage disequilibrium
It has been known for some time that the MHC comprises a large region in linkage disequilibrium (LD), or polymorphic frozen blocks (Dawkins et al. 1999 ). In some populations, for example, combinations of alleles, such as HLA-A1-B8-DR3, are more commonly found together than would be expected based on frequencies of individual alleles in the population. Several mechanisms may be invoked to explain this. First, there may have been insufficient time for recombination after relatively recent expansion of families, in some cases in isolated populations. Another possibility is that recombination ‘cold spots’ in the MHC sequence restrain the level of exchange of alleles between haplotypes. This may be facilitated by a reduced level of pairing at meiosis in a region such as the MHC, where the variation between haplotypes compromises homologous interaction. Indeed, overall recombination is low in the MHC.
An attractive explanation for the high LD is clustering of alleles that encode proteins that work well together and maintenance of functionally coordinated sets of alleles. As discussed above, the discovery of both TAP transporters and LMP proteasome components mapping to the MHC was largely serendipitous and was inspired to some extent by the notion that the MHC was a cluster of genes with inter-related functions. Furthermore, it was proposed that linkage of polymorphic transporters with polymorphic MHC class I genes permitted functional coordination, such that appropriate binding peptides, for the class I molecules linked in cis , were pumped by the relevant TAP. This turned out to be the case for rats and chickens, where genes for class I and TAP are in close proximity (Powis et al. 1992 ; Tregaskes et al. 2016 ). In the human MHC TAP transporters, genes are separated from the class I genes they serve by the class III region, so this functional integration may not be fully operational in our species. Another consideration is that each individual has two haplotypes, the various components of which must cooperate to some extent.
Recent data add weight to the notion of a high degree of allelic integration on individual haplotypes. It is becoming appreciated that, in addition to protein-coding loci, other polymorphic genomic features are embedded in the MHC complex. One clue to this came from the finding that a single nucleotide polymorphism (SNP) 35 kb upstream of HLA-C associated with levels of mRNA transcript and cell-surface expression (Kulkarni et al. 2011 ). Binding of microRNA hsa-miR-148 to a variable 3′ untranslated region in HLA-C genes regulated their expression. The conclusion was that expression levels may be regulated both by cis- and trans- acting factors. A further indication of the integration along haplotypes came from data relating to the way that class I molecules ‘educate’ by interacting with receptors on NK cells. Hydrophobic leader sequences from class I molecules supply peptides that bind HLA-E, which instructs CD94/NKG2A receptors on NK cells. Leader sequences of HLA-B molecules are dimorphic: Those with − 21 methionine (− 21 M) provide peptides that bind HLA-E, whereas − 21 threonine do not. Those haplotypes with -21 M rarely encode the ligands for other NK receptors, namely Bw4+ HLA-B and C2+ HLA-C. From these data, it was proposed that there are two schools of HLA haplotypes; one focused on supplying CD94/NKG2A ligands, and the other, KIR ligands. Individuals with − 21 M had NKG2A+ cells that were more effective than those with only − 21 T (Horowitz et al. 2016 ). Similarly, HLA-A leader sequences determine expression levels of HLA-E, again influencing interaction with CD94/NKG2A on NK cells and in turn HIV replication (Ramsuran et al. 2018 ).
Regulation of transcription and translation
Analysis of mutants has also been productive for identifying regulators of antigen processing and presenting genes. For many years, it was difficult to track down a master regulator of class I. On the other hand, mutants affecting class II were isolated from bare lymphocyte patients (Reith and Mach 2001 ). The class II master regulator CIITA is a nucleotide-binding domain and leucine-rich repeat receptor (NLR) protein. This large gene was initially found by expression cloning in a class II-deficient cell line (Steimle et al. 1993 ). Mutants invoking loss of function of CIITA generally result in severe immunodeficiency, a form of bare lymphocyte syndrome. Individuals with BLS have impaired antibody and T cell responses due to the lack of MHC class II expression. Class II transcription requires, in addition to CIITA, at least three additional factors: RFX5, RFX-AP and RFX-ANK. The genes encoding these factors were identified by comparing panels of rare BLS patients and mutant cell lines selected for loss of class II expression. This identified four complementation groups. Transient heterokaryon fusions between these cell lines restored class II expression if the partners harboured defects in different components, thereby defining the four groups. A combination of genetic complementation and protein characterisation eventually identified the genetic lesions, as reviewed in Reith and Mach ( 2001 ).
It was only in the last decade that a master transcription regulator for class I was discovered (Kobayashi and Elsen, 2012 ; Meissner et al. 2010 ; Neerincx et al. 2013 ). Like the class II transcription activator, CIITA, NLRC5 is an NLR protein and the two molecules share some homology. In cell lines defective in class I expression, such as mouse melanoma B16F10, over-expressed NLRC5 could restore class I expression. In human cells, over-expression of NLRC5 resulted in induction of non-classical class I genes HLA-E, -F and -G, which normally exhibit a restricted tissue expression. CIITA-dependent activation of HLA class II requires the enhanceosome complex, which binds to a motif, SXY, in the promoter of the gene. NLRCF probably interacts with a similar module upstream of class I genes.
Confirmation of the role of NLRC5 in regulation of MHC class I was provided by studying Nlrc5 -deficient knock-out mice (Staehli et al. 2012 ). The data showed that MHC class I was downregulated, exhibiting the greatest effect in cells in the immune system. However, treatment of these mice with inflammatory stimuli, such as interferon or LPS, resulted in significant class I expression, indicating that Nlrc5 -independent mechanisms must exist to regulate MHC class I.
Recent work suggests that there is still more to learn about variation in HLA expression. For example, the Anderson laboratory identified an elaborate system regulating forms of HLA-C, which is specific to natural killer cells, and could relate to NK cell licencing or education (Li et al. 2018 ). Other work suggests that, in addition to allelic differences, levels of class I should be taken into account in relation to autoimmunity, infection and transplantation (Apps et al. 2013 ; Kaur et al. 2017 ; Li et al. 2018 ; Petersdorf et al. 2014 ).
Screening for novel components
New screening techniques have the potential to identify novel factors involved in MHC biology. For example, forward genetic screens involving retroviral insertional mutagenesis or random CRISPR/Cas9 targeting of a haploid human cell line KBM7 implicate TXNDC11 as a novel factor required for MHC class I endoplasmic reticulum-associated protein degradation (ERAD) (Timms et al. 2016 ). In addition, a genome-wide RNAi screen was used to identify pathways regulating MHC class II antigen presentation (Paul et al. 2011 ).
Immunogenetics has played a predominant role in uncovering many of the fundamentals of antigen processing and presentation. In this review, we have sketched out some of the history and background to the genetics, focussing mainly on the human system. Other contributions to this volume of Immunogenetics may be consulted for more in-depth coverage of individual components of antigen processing and presentation.
https://www.ebi.ac.uk/cgi-bin/ipd/imgt/hla/fetch_cell.cgi?10972
Accolla RS (1983) Human B cell variants immunoselected against a single Ia antigen subset have lost expression of several Ia antigen subsets. J Exp Med 157:1053–1058
Article CAS PubMed Google Scholar
Apps R, Qi Y, Carlson JM, Chen H, Gao X, Thomas R, Yuki Y, Del Prete GQ, Goulder P, Brumme ZL, Brumme CJ, John M, Mallal S, Nelson G, Bosch R, Heckerman D, Stein JL, Soderberg KA, Moody MA, Denny TN, Zeng X, Fang J, Moffett A, Lifson JD, Goedert JJ, Buchbinder S, Kirk GD, Fellay J, McLaren P, Deeks SG, Pereyra F, Walker B, Michael NL, Weintrob A, Wolinsky S, Liao W, Carrington M (2013) Influence of HLA-C expression level on HIV control. Science 340:87–91
Article CAS PubMed PubMed Central Google Scholar
Badrinath S, Saunders P, Huyton T, Aufderbeck S, Hiller O, Blasczyk R, Bade-Doeding C (2012) Position 156 influences the peptide repertoire and tapasin dependency of human leukocyte antigen B*44 allotypes. Haematologica 97:98–106
Benacerraf B, McDevitt HO (1972) Histocompatibility-linked immune response genes. Science 175:273–279
Bjorkman PJ, Saper MA, Samraoui B, Bennett WS, Strominger JL, Wiley DC (1987a) The foreign antigen binding site and T cell recognition regions of class I histocompatibility antigens. Nature 329:512–518
Bjorkman PJ, Saper MA, Samraoui B, Bennett WS, Strominger JL, Wiley DC (1987b) Structure of the human class I histocompatibility antigen, HLA-A2. Nature 329:506–512
Blees A, Januliene D, Hofmann T, Koller N, Schmidt C, Trowitzsch S, Moeller A, Tampe R (2017) Structure of the human MHC-I peptide-loading complex. Nature 551:525–528
Bloom BR, Salgame P, Diamond B (1992) Revisiting and revising suppressor T cells. Immunol Today 13:131–136
Chen L, Fischer R, Peng Y, Reeves E, McHugh K, Ternette N, Hanke T, Dong T, Elliott T, Shastri N, Kollnberger S, James E, Kessler B, Bowness P (2014) Critical role of endoplasmic reticulum aminopeptidase 1 in determining the length and sequence of peptides bound and presented by HLA-B27. Arthritis Rheumatol 66:284–294
Cho SG, Attaya M, Monaco JJ (1991) New class II-like genes in the murine MHC. Nature 353:573–576
Dawkins R, Leelayuwat C, Gaudieri S, Tay G, Hui J, Cattley S, Martinez P, Kulski J (1999) Genomics of the major histocompatibility complex: haplotypes, duplication, retroviruses and disease. Immunol Rev 167:275–304
de la Salle H, Hanau D, Fricker D, Urlacher A, Kelly A, Salamero J, Powis SH, Donato L, Bausinger H, Laforet M et al (1994) Homozygous human TAP peptide transporter mutation in HLA class I deficiency. Science 265:237–241
Article PubMed Google Scholar
DeMars R, Chang CC, Shaw S, Reitnauer PJ, Sondel PM (1984) Homozygous deletions that simultaneously eliminate expressions of class I and class II antigens of EBV-transformed B-lymphoblastoid cells. I. Reduced proliferative responses of autologous and allogeneic T cells to mutant cells that have decreased expression of class II antigens. Hum Immunol 11:77–97
Denzin LK, Sant'Angelo DB, Hammond C, Surman MJ, Cresswell P (1997) Negative regulation by HLA-DO of MHC class II-restricted antigen processing. Science 278:106–109
Deverson EV, Gow IR, Coadwell WJ, Monaco JJ, Butcher GW, Howard JC (1990) MHC class II region encoding proteins related to the multidrug resistance family of transmembrane transporters. Nature 348:738–741
Garstka MA, Fritzsche S, Lenart I, Hein Z, Jankevicius G, Boyle LH, Elliott T, Trowsdale J, Antoniou AN, Zacharias M, Springer S (2011) Tapasin dependence of major histocompatibility complex class I molecules correlates with their conformational flexibility. FASEB J 25:3989–3998
Glynne R, Powis SH, Beck S, Kelly A, Kerr LA, Trowsdale J (1991) A proteasome-related gene between the two ABC transporter loci in the class II region of the human MHC. Nature 353:357–360
Goodfellow PN, Jones EA, Van Heyningen V, Solomon E, Bobrow M, Miggiano V, Bodmer WF (1975) The beta2-microglobulin gene is on chromosome 15 and not in the HL-A region. Nature 254:267–269
Green DR, Flood PM, Gershon RK (1983) Immunoregulatory T-cell pathways. Annu Rev Immunol 1:439–463
Guce AI, Mortimer SE, Yoon T, Painter CA, Jiang W, Mellins ED, Stern LJ (2013) HLA-DO acts as a substrate mimic to inhibit HLA-DM by a competitive mechanism. Nat Struct Mol Biol 20:90–98
Hermann C, van Hateren A, Trautwein N, Neerincx A, Duriez PJ, Stevanovic S, Trowsdale J, Deane JE, Elliott T, Boyle LH (2015) TAPBPR alters MHC class I peptide presentation by functioning as a peptide exchange catalyst. Elife 4
Horowitz A, Djaoud Z, Nemat-Gorgani N, Blokhuis J, Hilton HG, Beziat V, Malmberg KJ, Norman PJ, Guethlein LA, Parham P (2016) Class I HLA haplotypes form two schools that educate NK cells in different ways. Sci Immunol 1:eaag1672
Article PubMed PubMed Central Google Scholar
Horton R, Gibson R, Coggill P, Miretti M, Allcock RJ, Almeida J, Forbes S, Gilbert JG, Halls K, Harrow JL, Hart E, Howe K, Jackson DK, Palmer S, Roberts AN, Sims S, Stewart CA, Traherne JA, Trevanion S, Wilming L, Rogers J, de Jong PJ, Elliott JF, Sawcer S, Todd JA, Trowsdale J, Beck S (2008) Variation analysis and gene annotation of eight MHC haplotypes: the MHC haplotype project. Immunogenetics 60:1–18
Jiang J, Natarajan K, Boyd LF, Morozov GI, Mage MG, Margulies DH (2017) Crystal structure of a TAPBPR-MHC I complex reveals the mechanism of peptide editing in antigen presentation. Science 358:1064–1068
Karre K, Ljunggren HG, Piontek G, Kiessling R (1986) Selective rejection of H-2-deficient lymphoma variants suggests alternative immune defence strategy. Nature 319:675–678
Kasahara M (1999) The chromosomal duplication model of the major histocompatibility complex. Immunol Rev 167:17–32
Kaufman J (2015) Co-evolution with chicken class I genes. Immunol Rev 267:56–71
Kaur G, Gras S, Mobbs JI, Vivian JP, Cortes A, Barber T, Kuttikkatte SB, Jensen LT, Attfield KE, Dendrou CA, Carrington M, McVean G, Purcell AW, Rossjohn J, Fugger L (2017) Structural and regulatory diversity shape HLA-C protein expression levels. Nat Commun 8:15924
Kavathas P, Bach FH, DeMars R (1980) Gamma ray-induced loss of expression of HLA and glyoxalase I alleles in lymphoblastoid cells. Proc Natl Acad Sci U S A 77:4251–4255
Kelly A, Powis SH, Glynne R, Radley E, Beck S, Trowsdale J (1991a) Second proteasome-related gene in the human MHC class II region. Nature 353:667–668
Kelly A, Powis SH, Kerr LA, Mockridge I, Elliott T, Bastin J, Uchanska-Ziegler B, Ziegler A, Trowsdale J, Townsend A (1992) Assembly and function of the two ABC transporter proteins encoded in the human major histocompatibility complex. Nature 355:641–644
Kelly AP, Monaco JJ, Cho SG, Trowsdale J (1991b) A new human HLA class II-related locus, DM. Nature 353:571–573
Klein J (1986) Natural history of the major histocompatibility complex. Wiley, New York
Google Scholar
Kobayashi KS, van den Elsen PJ (2012) NLRC5: a key regulator of MHC class I-dependent immune responses. Nat Rev Immunol 12:813–820
Kronenberg M, Steinmetz M, Kobori J, Kraig E, Kapp JA, Pierce CW, Sorensen CM, Suzuki G, Tada T, Hood L (1983) RNA transcripts for I-J polypeptides are apparently not encoded between the I-A and I-E subregions of the murine major histocompatibility complex. Proc Natl Acad Sci U S A 80:5704–5708
Kulkarni S, Savan R, Qi Y, Gao X, Yuki Y, Bass SE, Martin MP, Hunt P, Deeks SG, Telenti A, Pereyra F, Goldstein D, Wolinsky S, Walker B, Young HA, Carrington M (2011) Differential microRNA regulation of HLA-C expression and its association with HIV control. Nature 472:495–498
Larhammar D, Hammerling U, Rask L, Peterson PA (1985) Sequence of gene and cDNA encoding murine major histocompatibility complex class II gene A beta 2. J Biol Chem 260:14111–14119
CAS PubMed Google Scholar
Li H, Ivarsson MA, Walker-Sperling VE, Subleski J, Johnson JK, Wright PW, Carrington M, Bjorkstrom NK, McVicar DW, Anderson SK (2018) Identification of an elaborate NK-specific system regulating HLA-C expression. PLoS Genet 14:e1007163
Liljedahl M, Kuwana T, Fung-Leung WP, Jackson MR, Peterson PA, Karlsson L (1996) HLA-DO is a lysosomal resident which requires association with HLA-DM for efficient intracellular transport. EMBO J 15:4817–4824
Meissner TB, Li A, Biswas A, Lee KH, Liu YJ, Bayir E, Iliopoulos D, van den Elsen PJ, Kobayashi KS (2010) NLR family member NLRC5 is a transcriptional regulator of MHC class I genes. Proc Natl Acad Sci U S A 107:13794–13799
Mellins E, Arp B, Ochs B, Erlich H, Pious D (1988) A single amino acid substitution in the human histocompatibility leukocyte antigen DR3 beta chain selectively alters antigen presentation. J Exp Med 168:1531–1537
Mellins E, Smith L, Arp B, Cotner T, Celis E, Pious D (1990) Defective processing and presentation of exogenous antigens in mutants with normal HLA class II genes. Nature 343:71–74
Monaco JJ, Cho S, Attaya M (1990) Transport protein genes in the murine MHC: possible implications for antigen processing. Science 250:1723–1726
Monaco JJ, McDevitt HO (1984) H-2-linked low-molecular weight polypeptide antigens assemble into an unusual macromolecular complex. Nature 309:797–799
Morris P, Shaman J, Attaya M, Amaya M, Goodman S, Bergman C, Monaco JJ, Mellins E (1994) An essential role for HLA-DM in antigen presentation by class II major histocompatibility molecules. Nature 368:551–554
Neerincx A, Boyle LH (2017) Properties of the tapasin homologue TAPBPR. Curr Opin Immunol 46:97–102
Neerincx A, Castro W, Guarda G, Kufer TA (2013) NLRC5, at the heart of antigen presentation. Front Immunol 4:397
Neerincx A, Hermann C, Antrobus R, van Hateren A, Cao H, Trautwein N, Stevanovic S, Elliott T, Deane JE, Boyle LH (2017) TAPBPR bridges UDP-glucose:glycoprotein glucosyltransferase 1 onto MHC class I to provide quality control in the antigen presentation pathway. Elife 6
Norman PJ, Hollenbach JA, Nemat-Gorgani N, Marin WM, Norberg SJ, Ashouri E, Jayaraman J, Wroblewski EE, Trowsdale J, Rajalingam R, Oksenberg JR, Chiaroni J, Guethlein LA, Traherne JA, Ronaghi M, Parham P (2016) Defining KIR and HLA class I genotypes at highest resolution via high-throughput sequencing. Am J Hum Genet 99:375–391
Ortmann B, Copeman J, Lehner PJ, Sadasivan B, Herberg JA, Grandea AG, Riddell SR, Tampe R, Spies T, Trowsdale J, Cresswell P (1997) A critical role for tapasin in the assembly and function of multimeric MHC class I-TAP complexes. Science 277:1306–1309
Park B, Lee S, Kim E, Ahn K (2003) A single polymorphic residue within the peptide-binding cleft of MHC class I molecules determines spectrum of tapasin dependence. J Immunol 170:961–968
Paul P, van den Hoorn T, Jongsma ML, Bakker MJ, Hengeveld R, Janssen L, Cresswell P, Egan DA, van Ham M, Ten Brinke A, Ovaa H, Beijersbergen RL, Kuijl C, Neefjes J (2011) A genome-wide multidimensional RNAi screen reveals pathways controlling MHC class II antigen presentation. Cell 145:268–283
Petersdorf EW, Gooley TA, Malkki M, Bacigalupo AP, Cesbron A, Du Toit E, Ehninger G, Egeland T, Fischer GF, Gervais T, Haagenson MD, Horowitz MM, Hsu K, Jindra P, Madrigal A, Oudshoorn M, Ringden O, Schroeder ML, Spellman SR, Tiercy JM, Velardi A, Witt CS, O'Huigin C, Apps R, Carrington M, International Histocompatibility Working Group in Hematopoietic Cell T (2014) HLA-C expression levels define permissible mismatches in hematopoietic cell transplantation. Blood 124:3996–4003
Pious D, Dixon L, Levine F, Cotner T, Johnson R (1985) HLA class II regulation and structure. Analysis with HLA-DR3 and HLA-DP point mutants. J Exp Med 162:1193–1207
Porter KM, Hermann C, Traherne JA, Boyle LH (2014) TAPBPR isoforms exhibit altered association with MHC class I. Immunology 142:289–299
Powis SJ, Deverson EV, Coadwell WJ, Ciruela A, Huskisson NS, Smith H, Butcher GW, Howard JC (1992) Effect of polymorphism of an MHC-linked transporter on the peptides assembled in a class I molecule. Nature 357:211–215
Powis SJ, Young LL, Joly E, Barker PJ, Richardson L, Brandt RP, Melief CJ, Howard JC, Butcher GW (1996) The rat cim effect: TAP allele-dependent changes in a class I MHC anchor motif and evidence against C-terminal trimming of peptides in the ER. Immunity 4:159–165
Ramsuran V, Naranbhai V, Horowitz A, Qi Y, Martin MP, Yuki Y, Gao X, Walker-Sperling V, Del Prete GQ, Schneider DK, Lifson JD, Fellay J, Deeks SG, Martin JN, Goedert JJ, Wolinsky SM, Michael NL, Kirk GD, Buchbinder S, Haas D, Ndung'u T, Goulder P, Parham P, Walker BD, Carlson JM, Carrington M (2018) Elevated HLA-A expression impairs HIV control through inhibition of NKG2A-expressing cells. Science 359:86–90
Reeves E, Colebatch-Bourn A, Elliott T, Edwards CJ, James E (2014a) Functionally distinct ERAP1 allotype combinations distinguish individuals with ankylosing spondylitis. Proc Natl Acad Sci U S A 111:17594–17599
Reeves E, Colebatch-Bourn A, Elliott T, Edwards CJ, James E (2015) Reply to Robinson and Brown: it is the combination of ERAP1 allotypes that identifies individuals with ankylosing spondylitis. Proc Natl Acad Sci U S A 112:E1817
Reeves E, Elliott T, James E, Edwards CJ (2014b) ERAP1 in the pathogenesis of ankylosing spondylitis. Immunol Res 60:257–269
Reith W, Mach B (2001) The bare lymphocyte syndrome and the regulation of MHC expression. Annu Rev Immunol 19:331–373
Robinson PC, Brown MA (2015) ERAP1 biology and assessment in ankylosing spondylitis. Proc Natl Acad Sci U S A 112:E1816
Sakaguchi S, Wing K, Miyara M (2007) Regulatory T cells—a brief history and perspective. Eur J Immunol 37(Suppl 1):S116–S123
Salter RD, Howell DN, Cresswell P (1985) Genes regulating HLA class I antigen expression in T-B lymphoblast hybrids. Immunogenetics 21:235–246
Shiina T, Inoko H, Kulski JK (2004) An update of the HLA genomic region, locus information and disease associations: 2004. Tissue Antigens 64:631–649
Spies T, Bresnahan M, Bahram S, Arnold D, Blanck G, Mellins E, Pious D, DeMars R (1990) A gene in the human major histocompatibility complex class II region controlling the class I antigen presentation pathway. Nature 348:744–747
Spies T, DeMars R (1991) Restored expression of major histocompatibility class I molecules by gene transfer of a putative peptide transporter. Nature 351:323–324
Spring B, Fonatsch C, Muller C, Pawelec G, Kompf J, Wernet P, Ziegler A (1985) Refinement of HLA gene mapping with induced B-cell line mutants. Immunogenetics 21:277–291
Staehli F, Ludigs K, Heinz LX, Seguin-Estevez Q, Ferrero I, Braun M, Schroder K, Rebsamen M, Tardivel A, Mattmann C, MacDonald HR, Romero P, Reith W, Guarda G, Tschopp J (2012) NLRC5 deficiency selectively impairs MHC class I-dependent lymphocyte killing by cytotoxic T cells. J Immunol 188:3820–3828
Steimle V, Otten LA, Zufferey M, Mach B (1993) Complementation cloning of an MHC class II transactivator mutated in hereditary MHC class II deficiency (or bare lymphocyte syndrome). Cell 75:135–146
Steinmetz M, Hood L (1983) Genes of the major histocompatibility complex in mouse and man. Science 222:727–733
Teng MS, Stephens R, Du Pasquier L, Freeman T, Lindquist JA, Trowsdale J (2002) A human TAPBP (TAPASIN)-related gene, TAPBP-R. Eur J Immunol 32:1059–1068
Timms RT, Menzies SA, Tchasovnikarova IA, Christensen LC, Williamson JC, Antrobus R, Dougan G, Ellgaard L, Lehner PJ (2016) Genetic dissection of mammalian ERAD through comparative haploid and CRISPR forward genetic screens. Nat Commun 7:11786
Tonnelle C, DeMars R, Long EO (1985) DO beta: a new beta chain gene in HLA-D with a distinct regulation of expression. EMBO J 4:2839–2847
Townsend A, Ohlen C, Bastin J, Ljunggren HG, Foster L, Karre K (1989) Association of class I major histocompatibility heavy and light chains induced by viral peptides. Nature 340:443–448
Townsend AR, Gotch FM, Davey J (1985) Cytotoxic T cells recognize fragments of the influenza nucleoprotein. Cell 42:457–467
Tregaskes CA, Harrison M, Sowa AK, van Hateren A, Hunt LG, Vainio O, Kaufman J (2016) Surface expression, peptide repertoire, and thermostability of chicken class I molecules correlate with peptide transporter specificity. Proc Natl Acad Sci U S A 113:692–697
Trowsdale J, Hanson I, Mockridge I, Beck S, Townsend A, Kelly A (1990) Sequences encoded in the class II region of the MHC related to the ‘ABC’ superfamily of transporters. Nature 348:741–744
Trowsdale J, Kelly A (1985) The human HLA class II alpha chain gene DZ alpha is distinct from genes in the DP, DQ and DR subregions. EMBO J 4:2231–2237
Turner TR, Hayhurst JD, Hayward DR, Bultitude WP, Barker DJ, Robinson J, Madrigal JA, Mayor NP, Marsh SGE (2018) Single molecule real-time DNA sequencing of HLA genes at ultra-high resolution from 126 international HLA and immunogenetics workshop cell lines. HLA 91:88–101
van Ham SM, Tjin EP, Lillemeier BF, Gruneberg U, van Meijgaarden KE, Pastoors L, Verwoerd D, Tulp A, Canas B, Rahman D, Ottenhoff TH, Pappin DJ, Trowsdale J, Neefjes J (1997) HLA-DO is a negative modulator of HLA-DM-mediated MHC class II peptide loading. Curr Biol 7:950–957
Williams AP, Peh CA, Purcell AW, McCluskey J, Elliott T (2002) Optimization of the MHC class I peptide cargo is dependent on tapasin. Immunity 16:509–520
Zimmer J, Andres E, Donato L, Hanau D, Hentges F, de la Salle H (2005) Clinical and immunological aspects of HLA class I deficiency. QJM 98:719–727
Zinkernagel RM, Doherty PC (1974) Immunological surveillance against altered self components by sensitised T lymphocytes in lymphocytic choriomeningitis. Nature 251:547–548
Download references
JT is supported by the European Research Council under the European Union’s Horizon 2020 research and innovation programme (Grant agreement No. 695551).
Author information
Authors and affiliations.
Department of Pathology, University of Cambridge, Cambridge, CB21QP, UK
Adrian Kelly & John Trowsdale
You can also search for this author in PubMed Google Scholar
Corresponding author
Correspondence to John Trowsdale .
Additional information
This article is part of the Topical Collection on Biology and Evolution of Antigen Presentation
Rights and permissions
Open Access This article is distributed under the terms of the Creative Commons Attribution 4.0 International License (http://creativecommons.org/licenses/by/4.0/), which permits unrestricted use, distribution, and reproduction in any medium, provided you give appropriate credit to the original author(s) and the source, provide a link to the Creative Commons license, and indicate if changes were made.
Reprints and permissions
About this article
Kelly, A., Trowsdale, J. Genetics of antigen processing and presentation. Immunogenetics 71 , 161–170 (2019). https://doi.org/10.1007/s00251-018-1082-2
Download citation
Received : 02 August 2018
Accepted : 24 August 2018
Published : 13 September 2018
Issue Date : 01 March 2019
DOI : https://doi.org/10.1007/s00251-018-1082-2
Share this article
Anyone you share the following link with will be able to read this content:
Sorry, a shareable link is not currently available for this article.
Provided by the Springer Nature SharedIt content-sharing initiative
- Antigen processing
- Antigen presentation
- Find a journal
- Publish with us
- Track your research
- Open access
- Published: 02 June 2024
Adjuvants for cancer mRNA vaccines in the era of nanotechnology: strategies, applications, and future directions
- Lei-Ming Cao 1 na1 ,
- Yi-Fu Yu 1 na1 ,
- Zi-Zhan Li 1 ,
- Nian-Nian Zhong 1 ,
- Guang-Rui Wang 1 ,
- Yao Xiao 1 ,
- Bing Liu 1 , 2 ,
- Qiu-Ji Wu 3 ,
- Chun Feng 4 &
- Lin-Lin Bu 1 , 2
Journal of Nanobiotechnology volume 22 , Article number: 308 ( 2024 ) Cite this article
17 Accesses
1 Altmetric
Metrics details
Research into mRNA vaccines is advancing rapidly, with proven efficacy against coronavirus disease 2019 and promising therapeutic potential against a variety of solid tumors. Adjuvants, critical components of mRNA vaccines, significantly enhance vaccine effectiveness and are integral to numerous mRNA vaccine formulations. However, the development and selection of adjuvant platforms are still in their nascent stages, and the mechanisms of many adjuvants remain poorly understood. Additionally, the immunostimulatory capabilities of certain novel drug delivery systems (DDS) challenge the traditional definition of adjuvants, suggesting that a revision of this concept is necessary. This review offers a comprehensive exploration of the mechanisms and applications of adjuvants and self-adjuvant DDS. It thoroughly addresses existing issues mentioned above and details three main challenges of immune-related adverse event, unclear mechanisms, and unsatisfactory outcomes in old age group in the design and practical application of cancer mRNA vaccine adjuvants. Ultimately, this review proposes three optimization strategies which consists of exploring the mechanisms of adjuvant, optimizing DDS, and improving route of administration to improve effectiveness and application of adjuvants and self-adjuvant DDS.
Introduction
Since the outbreak of coronavirus disease 2019 (COVID-19) in early 2020, severe acute respiratory syndrome coronavirus 2 has spread globally, resulting in over 250 million confirmed cases [ 1 ]. In the fight against COVID-19, mRNA vaccines have emerged as a prominent solution. These vaccines offer significant advantages over traditional vaccine technologies, including higher production efficiency and enhanced safety. Moderna, a leading entity among mRNA vaccine developers, rapidly identified the antigenic sequence of the virus and produced the first mRNA-1273 vaccine within just 45 days. This vaccine later demonstrated a 94.1% efficacy rate in a phase III clinical trial, underscoring the promising potential of mRNA vaccine technology for future infectious disease responses [ 2 ]. The mRNA vaccine has emerged as a vital tool in humanity’s arsenal against the novel coronavirus. Beyond their application in viral infections, mRNA vaccines are also being explored for their potential in cancer treatment. Several clinical trials involving mRNA-based cancer vaccines have yielded promising outcomes, highlighting the potential of mRNA vaccines in oncology. This development points to a broader scope of application for mRNA technology, potentially revolutionizing the approach to cancer treatment [ 3 , 4 ].
The remarkable success of mRNA vaccines can be attributed to several key advantages, we summarized them by the acronym “ WESP ” (Fig. 1 ): (1) Wide applicability. mRNA vaccines can encode almost any protein and facilitate post-translational modifications within cells. This capability reduces immunogenicity while ensuring the functionality of protein products, leading to significant breakthroughs in treating various diseases [ 5 , 6 , 7 ]. (2) Efficiency . Appropriate modification and optimization of sequence can significantly improve mRNA stability and translation efficiency. Currently, there is already an efficient drug delivery systems (DDS) that can achieve rapid uptake and cytoplasmic expression of mRNAs [ 8 , 9 , 10 ]. (3) Safety . Unlike DNA vaccines, the mRNA platform is non-infectious and non-integrating, eliminating the risk of infection or gene insertion [ 11 ]. (4) Productiveness . Once the genome sequence of a pathogen is known, mRNA encoding the antigenic protein can be swiftly designed and produced. This was exemplified by the rapid development of mRNA vaccines for COVID-19. Furthermore, the high yield from in vitro transcription not only ensures rapid production but also makes the process cost-effective and scalable [ 2 ].
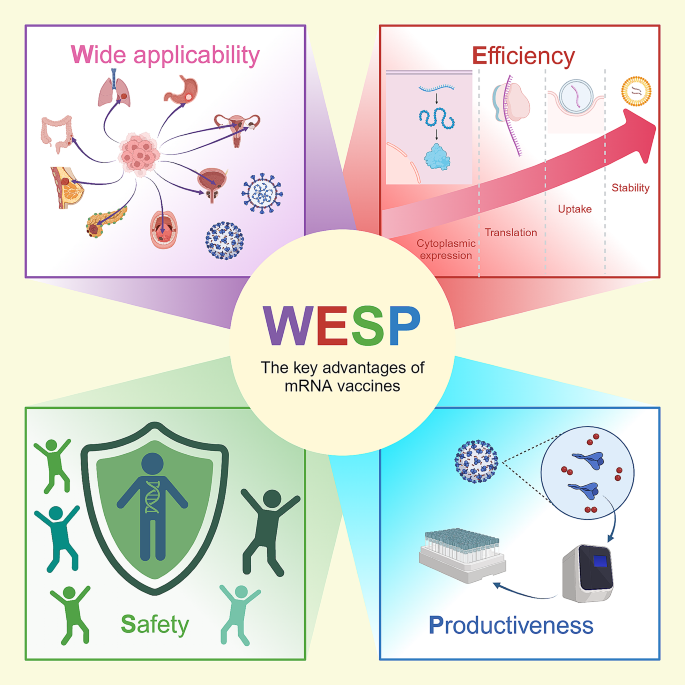
“WESP”-the key advantages of mRNA vaccines. The remarkable success of mRNA vaccines can be attributed to several key advantages which can be summarized by the acronym “WESP”: “W”: Wide applicability, “E”: Efficiency, “S”: Safety, “P”: Productiveness
Aforementioned attributes collectively underscore the effectiveness and potential of mRNA vaccines as a pivotal tool in modern medicine, capable of addressing both infectious diseases and complex conditions like cancer. The mRNA vaccines offer a promising approach to cancer treatment by their ability to encode tumor-related antigens and elicit an immune response. The core principle of mRNA cancer vaccines involves transporting transcripts that encode for tumor-associated antigens or tumor-specific antigens into the cytoplasm of host cells, particularly antigen-presenting cells (APCs). This capability allows the immune system to recognize and target cancer cells effectively, potentially transforming cancer therapy by providing a highly specific and adaptive treatment option [ 12 , 13 , 14 ]. Currently, mRNA cancer vaccines made significant achievements in the treatment of prostate cancer. The prostate cancer vaccines CV9103, developed by Curevacs (Germany), has already undergone phase I/II clinical trials, during which it was demonstrated to be well tolerated and to elicit a favorable immune-activation [ 15 ]. In addition, in the phase I trial of a novel personalized mRNA neoantigen vaccine, it stimulated high-magnitude neoantigen-specific and long-lived polyfunctional CD8 + T cells in pancreatic cancer, resulting in a longer recurrence-free survival [ 16 ]. To date, mRNA vaccines have made notable achievements in the field of cancer treatment.
However, mRNA vaccines still face several challenges. The primary concern is their instability and inability to penetrate the physiological barriers in human body, which prevents them from reaching target cells [ 17 ]. In the human body, mRNAs are susceptible to degradation by RNases or recognition and phagocytosis by macrophages or dendritic cells (DCs) in the liver [ 1 ]. While the naked mRNA can still be taken up by the cell, the process is too inefficient. To increase effectiveness, repeated administrations are required [ 17 ]. Nevertheless, excessive amounts of drug can lead to immune-related adverse reactions [ 18 ]. In addition, the mRNA vaccine (BNT162b2) administered by intramuscularly injection was mainly distributed in the site of injection and the liver, resulting in reversible liver damage in animals [ 19 ]. Therefore, it is challenging to achieve specific organ targeting for mRNA cancer vaccines.
To cope with these challenges, the design and selection of adjuvants is crucial. Adjuvants have the ability to enhance body’s immune response, optimize drug delivery routes, reduce drug toxicity, and enhance drug efficacy by precisely targeting and reducing the total drug volume. However, the definition of adjuvant remains controversial. According to the traditional view, an mRNA vaccine comprises three components: mRNA sequence containing antigen, DDS or vector, and adjuvant. Adjuvant is an immunostimulant that is added in addition to a vector or DDS to non-specifically enhance the body’s specific immune response to the antigen [ 7 ]. Conversely, some scholars argue that adjuvants should include DDS in addition to traditional adjuvants [ 20 ]. This is because some self-adjuvant delivery materials, such as mesoporous silicon rods, have the ability to enhance the strength, breadth and durability of the immune response itself [ 21 ]. In this review, we will discuss immunostimulants or DDS that have an immunopotentiation effect on mRNA vaccines, all of which will be considered as adjuvants. The lack of a systematic overview and summary of the mechanism of action, combined with the complexity of the mechanism and the broad definition of adjuvant, has caused inconvenience and confusion for researchers in designing appropriate vaccine adjuvants [ 20 ]. To aid researchers in comprehending adjuvants of mRNA vaccines, this review will describe the design strategies for adjuvants, introduce their mechanisms, and summarize the limitations and side effects of existing adjuvants, and provide prospects for future improvement.
Design strategies for immunostimulants
Immunostimulants, often recognized as danger signal molecules, function as pathogen-associated molecular patterns (PAMPs), damage-associated molecular patterns, or their mimics. These substances are pivotal in triggering the innate immune response. They achieve this by targeting pattern recognition receptors (PRRs) on APCs. Upon activation, APCs undergo a maturation process during which their antigen phagocytic activity ceases, and their capability to present antigens is enhanced. Concurrently, these matured APCs express higher levels of co-stimulatory signals and cytokines, which are crucial for initiating and amplifying adaptive immune responses [ 22 ]. Currently, the design strategies for immunostimulants are targeting different PRRs to lead different cytokine secretion [ 23 ]. Based on the different targeting pathway, there are four dominant design strategies for immunostimulants. Besides, there is a special design strategy for immunostimulants, which is using cytokines as immunostimulants (Fig. 2 ).

The design strategies for immunostimulants. Various types of immunostimulants activate different PRRs, leading to the secretion of various cytokines and inducing diverse adaptive immune responses. Immunostimulants activate TLRs, cGAS-STING, CLRs, other PRRs, or directly release cytokines to induce and modulate adaptive immune responses. Binding to TLRs heterodimers initiates MyD88 pathway and activated NF-κB and ERK1/2 to enhance pro-inflammatory cytokines. The mtDNA and dsDNA initiates the conversion of cGAS into cGAMP and consequently activates STING to release TBK to activates IRF 3 and IKKi to activates NF-κB. Finally, IRF 3 induces type 1 interferons, cross presentation and CTL and NF-κB induces pro-inflammatory cytokines and activate Th1 cells. Targeting to most CLRs activated NF-κB to enhance pro-inflammatory cytokines. Notably, targeting to CD205 and CD206 can enhance endocytosis and antigen presentation. Common immunostimulants targeting to NLRs (NOD 1 and NOD 2) activated NF-κB ultimately produces a predominantly Th2-type of immune response. Alternatively, immunostimulants targeting to MDA5 and RIG-I activate IRF 3 and IRF 7 respectively. At length, IRF 3 and IRF 7 induce type 1 interferons, cross presentation, CTL and activate Th1 cells
Targeting TLRs pathway
Immunostimulants can enhance antigen presentation and upregulate costimulatory signals and cytokine expression by targeting Toll-like receptors (TLRs) on APCs, ultimately enhancing the adaptive immune response [ 24 , 25 , 26 , 27 , 28 ]. One classical mechanism of action for immunostimulants involves their binding to TLRs heterodimers, specifically TLR2/1 or TLR2/6. This interaction initiates signaling through the myeloid differentiation primary response 88 (MyD88) pathway. Subsequent to this signaling event, the transcription factor nuclear factor kappa-light-chain-enhancer of activated B cells (NF-κB) is activated. NF-κB activation leads to the production of pro-inflammatory cytokines, which play a critical role in the differentiation of naive T cells into T helper 1 cells [ 29 , 30 ]. Simultaneously, the activation of the extracellular signal-regulated kinase 1/2 (ERK1/2) pathway enhances the signaling cascade, leading to increased expression of the c-Fos protein. This increase in c-Fos levels plays a pivotal role in modulating cytokine expression; specifically, it enhances the production of interleukin-10 (IL-10) and suppresses the expression of interleukin-12 (IL-12). This drives the conversion of naive T cells into Th2-type cells [ 31 ]. Consequently, immunostimulants targeting TLR2 primarily induce Th2-type adaptive immune response. Here are some common immunostimulants that target TLRs: lipopolysaccharide (LPS), monophosphoryl lipid (MPL), cytosine phospho-guanosine oligonucleotides [CpG (TLR9a)] and R848. LPS, a potent TLR4 agonist, is a natural immune adjuvant from the outer membrane of Gram-negative bacteria [ 32 ]. MPL contains the adjuvant active principle of LPS (lipid A) [ 33 ]. CpG (TLR9a), an agonist of TLR9, has been widely used as an adjuvant in mRNA cancer vaccine. R848 is recognized by TLR7 and TLR8. The immune cells like monocytes and macrophages are activated by TLR7 and TLR8 and then secrete cytokines to mediate innate and adaptive immune responses [ 34 ].
Targeting cGAS-STING pathway
The cyclic guanosine monophosphate (GMP)-adenosine monophosphate (AMP) synthase (cGAS) functions as a cytoplasmic DNA sensor that is activated by the presence of double-stranded DNA (dsDNA) and mitochondrial DNA (mtDNA). Upon activation by such DNA, cGAS catalyzes the conversion of cytoplasmic AMP and GMP into cyclic guanosine monophosphate-adenosine monophosphate (cGAMP) [ 35 ]. Subsequently, cGAMP aggregates and activates STING through conformational change, which then activates NF-κB and interferon regulatory factor 3 (IRF 3), promoting the production of type I interferons and pro-inflammatory cytokines. Finally, the APCs will have a terrific ability to present or cross-present antigens [ 36 , 37 ]. Immunostimulants that target the cGAS-STING pathway include nucleotide and non-nucleotide small molecule agonists. The former are mainly natural ligand molecules which based on cyclic dinucleotides. For instance, the cyclic dimeric adenosine monophosphate, cyclic dimeric guanosine monophosphate, 3’,3’-cGAMP, and 2’,3’-cGAMP [ 38 ]. Examples of common non-nucleotide small molecule agonists include CF 501 and DMXAA [ 39 , 40 ].
Targeting CLRs pathway
The C-type lectin receptors (CLRs) superfamily comprises various receptors, such as MINCLE, DC-SIGN, Dectin-1, Dectin-2, CD205, CD206, and others. CLRs are primarily located on cell membranes and act as antigen receptors for capturing and presenting antigens [ 41 , 42 ]. In most instances, immunostimulants that have a carbohydrate structure can activate the CLRs and stimulate the APCs to initiate the internalization, presentation and processing of antigens, thereby enhancing the adaptive immune response [ 43 , 44 ]. Comparatively, there has been less research on the potential of immunostimulants targeting CLRs pathway. However, it has been found that fungal mannans can act as immune adjuvants, which can elicit a potent antigen-specific neutralizing antibodies to increase the immune response and can be harnessed for vaccine [ 45 ]. Immunostimulants that targeted CLRs pathway has a great deal of untapped potential.
Targeting other PRRs
In addition to the three major pathways mentioned above, the nucleotide-binding oligomerization domain-like receptors (NLRs) family which includes nucleotide-binding oligomerization domain 1 (NOD1), nucleotide-binding oligomerization structural domain 2 (NOD2), and NOD-like receptor thermal protein domain associated protein 3 (NLRP 3), can also be targeted [ 46 ]. Common immunostimulants that target NLRs are muramyl dipeptide and complete Freund’s adjuvant, which ultimately produces a predominantly Th2-type of immune response [ 28 ]. Furthermore, retinoic acid-inducible gene I-like receptors family can also be targeted as they primarily recognize RNA. The main members of this family are melanoma differentiation-associated gene 5 (MDA5) and retinoic acid-induced gene I (RIG-I) [ 47 ].
Cytokine immunostimulant
In addition to the immunostimulants mentioned above, there is a distinct category of immunostimulants called cytokines. Cytokines are small soluble polypeptide proteins secreted by immune and non-immune cells under certain conditions. They play a regulatory role intercellularly and intracellularly. Their effectiveness as adjuvants highly depends on the dose, form, route of administration, and the type of co-administered vaccine [ 48 ]. Interleukin-2 (IL-2) and granulocyte-macrophage colony stimulating factor (GM-CSF) are two mature cytokine immunostimulant. GM-CSF promotes the maturation and activation of APCs and IL-2 enhances immune response of T cells [ 49 ]. Interferon-alpha (IFN-alpha) and tumor necrosis factor (TNF) are also being investigated for their potential adjuvant effects. They enhance the immunoregulatory function of natural killer cells, promote the differentiation of T lymphocytes and play a broad up-regulatory role in the body’s immune response [ 50 ].
Application of immunostimulant in mRNA cancer vaccines.
The current applications of immunostimulant in mRNA cancer vaccines are as follows: (1) Protamine. Arginine-rich protamine peptides have been demonstrated to form a complex with mRNA, subsequently activating TLR7/8 pathways to elicit T-cell and B-cell-dependent immune responses against non-small-cell lung cancer, prostate cancer, and melanoma [ 51 , 52 , 53 , 54 ]. (2) DP7. The cationic peptide DP7 with cholesterol-modified (VQWRIR-VAVIRK) activates the TLR2-MyD88-IKK-NF-κB pathway and enhance the immune responses stimulated by the mRNA cancer vaccine. Notably, DP7 has been identified as an effective immunostimulant for personalized mRNA cancer vaccines [ 55 ]. (3) R848. The TLR7/8 agonist R848 modified with palmitic acid (C16-R848) has been demonstrated to effectively activate the adaptive immune response and to enhance the delivery efficiency of the mRNA cancer vaccine in prostate and lymphoma tumor model mice [ 56 ]. (4) α-galactosylceramide (α-GC). The α-GC is a glycolipid antigen that can be presented in the CD1d, the MHC-I-like molecule on APCs, to stimulate invariant natural killer T cells and evoke pluripotent innate and adaptive antitumor immune response in lymphoma animal models [ 57 ].
Design strategies for self-adjuvant drug delivery systems for mRNA vaccines
The definition of DDS is carrier materials that load antigen and increase the ability of APCs to uptake and present antigen [ 20 ]. The main function of DDS for mRNA vaccines is to aid in antigen presentation by assisting mRNAs in crossing the three barriers of the body: the extracellular barrier, lysosomal escape, and intracellular immunity. This results in an increase in the antigenic signals on the surface of APCs [ 1 ]. Notably, the precise DDS for mRNA vaccines can reduce the toxicity of the vaccine, reduce the total amount of drug, and increase the efficacy of the vaccine. Currently, there are two main types of delivery vectors: viral vectors and non-viral vectors. Although viral vectors have the advantage of high transfection efficiency, the safety concerns remain a significant issue. The enthusiasm for viral vector research has largely waned after two clinical trials in which the use of viral vectors resulted in the deaths of volunteers [ 58 , 59 ]. Attention has shifted to non-viral vectors due to the stagnation of viral vector research. Non-viral vectors, with their low toxicity and low immunogenicity, have become one of the hottest research directions at present. Nevertheless, drugs delivered by non-viral vectors also have the disadvantage of low escape efficiency in nuclear endosomes or lysosomes and weak ability to target to cells, tissues, and organs [ 60 , 61 ]. There is an urgent need for novel non-viral DDS for mRNA vaccines to overcome these difficulties. There are four common strategies (Fig. 3 ):
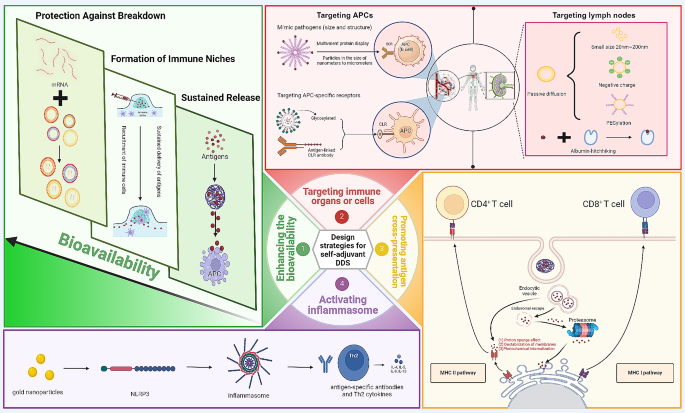
The design strategies for self-adjuvant drug delivery systems. Self-adjuvant delivery systems increased antigen presentation to enhance adaptive immune responses by enhancing the bioavailability of antigens; targeting immune organs or cells; promoting antigen cross-presentation; and activating inflammasome. Enhancing the bioavailability of antigens can be achieved by sustained releasing antigens, formatting immune niches, and protecting antigens from breakdown. Targeting APCs can be achieved by using nanoscale materials, constructing highly ordered and repetitive spatial structures to mimic pathogens and targeting specific receptors on APCs. Targeting lymph node can achieved through the design of suitable dimensions (20 to 200 nm), surface properties (negative charge and hydrophobicity) and albumin-hitchhiking. Promoting antigen cross-presentation can be enabled in three main ways, proton sponge effect, destabilization of membranes and photochemical internalization
Enhancing the bioavailability of antigens
(1) Sustained Release : By prolonging the presence of antigens within the immune system through their sustained release, there is a prolonged opportunity for immune system interaction. This method ensures that antigens are continuously available to stimulate an immune response [ 62 ]. (2) Formation of Immune Niches : Creating immune niches at the site of injection can recruit additional immune cells. This influx of immune cells enhances antigen uptake and activates the adaptive immune response, thereby stimulating the release of cytokines and chemokines. This process intensifies the immune system’s engagement with the antigen [ 63 ]. (3) Protection Against Breakdown : Protecting antigen from breakdown by mRNA enzymes and slowing down the antigen digestion process are crucial, which is one of the benefits of lipid nano-particle (LNP) [ 4 , 64 , 65 ].
Targeting immune organs or cells
Targeting APCs in the immune microenvironment can enhance immune presentation and phagocytosis. Several methods can be employed to achieve this: (1) Using microscale or nanoscale materials to adjust the dimension of antigen to mimic pathogens [ 66 ]. (2) Constructing highly ordered and repetitive spatial structures similar to those inherent in pathogens allows the immune system to recognize and respond to these structural features with greater sensitivity [ 67 ]. Furthermore, these structures can facilitate the co-aggregation of B cell receptor (BCR) and the eventually produce high-affinity antibodies and memory B cells [ 68 , 69 ]. (3) Targeting the specific receptors on APCs, such as Fc receptors [ 70 , 71 , 72 , 73 ].
Lymph node metastasis is a significant prognostic factor that signals a worse prognosis and reflects the necessity of systemic therapy in the majority of cancer patients [ 74 ]. For patients with oral squamous cell carcinomas, the five-year survival rate can decline to below 20% when lymph node metastasis occur [ 75 ]. Consequently, targeting the mRNA vaccines to lymph nodes is an ideal design strategy for DDS. The precise delivery of mRNA vaccine to lymph nodes can change the pharmacokinetics, activate a long-lasting and potent immune response, and reduce undesired systemic toxicity and side effects [ 76 , 77 ]. In addition, targeting lymph nodes can significantly augment the innate immune response, particularly by activating macrophages within the lymph nodes, which in turn enhances the anti-tumor efficacy of mRNA vaccines [ 78 ]. The high anti-tumor efficacy of the lymph node-targeting DDS demonstrates considerable potential as a design strategy for mRNA vaccines [ 77 ].
Effective strategies to achieve this include: (1) Designing a DDS for mRNA vaccines of suitable dimensions (20 to 200 nm) and surface properties (net negative charge and hydrophobicity) that relies on passive diffusion to enter the afferent lymphatics and subsequently enter lymph nodes [ 79 , 80 , 81 ]. (2) Albumin-hitchhiking, which exploits the ability of endogenous albumin to circulate in the lymphatic system. Binding the antigen to endogenous albumin, thereby antigen is transported to the lymph nodes via the albumin train [ 82 , 83 ]. Notably, this method is found to be highly effective in inhibiting the growth of primary or metastatic tumors in mice [ 84 ].
Alternatively, in LNP, there is a special target needs to be considered cautiously, the non-liver tissues target [ 85 ]. LNP is a mature technique for the delivery of genetic medicines. However, its therapeutic application is limited due to the liver accumulation. The apolipoprotein E in serum binds to LNP and causes mRNA to preferentially enter the liver, which produces enzymes that interfere with the effectiveness of the mRNA vaccine, preventing the LNP@mRNA from achieving its full potential [ 86 ]. In order to address liver accumulation, the addition of the selective organ targeting (SORT) lipids can achieve specific targeting of organs such as the liver, lungs, spleen, etc., thus enabling non-liver tissues target [ 85 ].
Promoting antigen cross-presentation
In most cases, exogenous antigens are just internalized by APCs and only presented to CD4 + T cells by major histocompatibility complex II (MHC II) molecules, without any cross-presentation which is the process by which exogenous antigens are presented to CD8 + T cells by major histocompatibility complex I (MHC I) molecules [ 87 , 88 ]. This type of presentation elicits a weak immune response. To increase the strength of the immune response, some DDS for mRNA vaccines have enabled antigen cross-presentation by facilitating the escape of antigens from lysosomes or endosomes [ 20 ]. Cross-presentation can be enabled in three main ways: (1) Proton sponge effect. When some DDS for mRNA vaccines containing protonable amine groups are internalized by the APCs, substantial protons are absorbed by the APCs. To neutralize the acidic environment of the lysosome or endosomal of APCs, chloride ions and water will flow from the cytoplasm into endosomes or lysosomes in large amounts, which causes swelling and rupture of the endosomes. Subsequently, the antigens are released into the cytoplasm, which facilitates the cross-presentation of the antigen by MHC I molecules [ 89 ]. (2) Binding or fusing to the membranes of endosomal or lysosomal. This process destabilizes the endosomal/lysosomal membrane, releasing the antigen into the cytoplasm, which facilitates the cross-presentation of the antigen by MHC I molecules [ 90 ]. (3) Photochemical internalization release technology. This is an emerging technology that uses photosensitizers to release antigens into the cytoplasm through light-induced disruption of endosomal membranes [ 91 , 92 , 93 ].
Activating inflammasome
Inflammasome, multi-protein complexes assembled with the participation of PRRs, is an important component of the innate immune system [ 94 ]. Gold nanoparticles, one of the most mature inorganic nano-drug delivery systems, can promote the production of antigen-specific antibodies and Th2 cytokines through the activation of NLRP3 inflammasome, in addition to the protection of antigens from hydrolysis by mRNA enzymes and targeting to the lymph nodes mentioned above [ 95 ].
Application of self-adjuvant DDS in mRNA cancer vaccines
Currently, the applications of self-adjuvant DDS in mRNA cancer vaccines are listed as follows: (1) LNP. The use of endogenously LN-targeting LNP can improve the effectiveness of mRNA vaccine by stimulating robust humoral responses and T follicular helper cell [ 96 ]. The 113-O12B is an effective LN-targeting DDS for mRNA cancer vaccines and can improve the effectiveness of anti-tumour treatment [ 77 ]. The BNT-113, an mRNA vaccine encapsulated within LNP, has demonstrated encouraging efficacy against head and neck cancer. It is currently undergoing phase II clinical trials (NCT04534205) [ 97 ]. In addition, the mRNA-4157 is a personalized mRNA vaccine encapsulated in LNP too. It can encode multiple neoantigens, thereby inducing neoantigen-specific T cells and eliciting anti-tumor immune responses in patients with head and neck cancer [ 98 ]. Furthermore, loading comb-structured mRNA, which consists of antigen-producing single-stranded mRNA, and adjuvant short double-stranded RNA, onto LNP enables immunostimulation in different formulations of mRNA cancer vaccines [ 99 ]. The mRNA vaccine combining all-trans-retinoic acid with LNP has shown significant tumor inhibition effects in animal model for the treatment of orthotopic colorectal tumors [ 100 ]. (2) Polyguanidine (PolyGu). Branched PolyGu nanovaccines are used to integrate immunostimulant functions into the DDS, resulting in self-adjuvating PolyGu nanovaccines. It can effectively stimulate and promoted the maturation of DCs through TLR4 and NLRP3 pathways, and exhibited strong immune activity in vivo. In addition, PolyGu can improve the delivery efficiency of mRNA as a DDS and effectively suppress tumour growth, thereby prolonging the survival of mice [ 101 ]. . (3) Self-assembled RNA origami (RNA-OG). The RNA-OG nanostructure functions as a TLR 3 agonist and is a suitable DDS for mRNA cancer vaccines due to its versatility in modification and robust synthesis. Studies have shown that the assembled RNA-OG-peptide nanovaccines induce DCs maturation, mobilize tumor-specific CD8 + T cell responses, and reduce tumor-mediated immunosuppression [ 102 ]. In the field of colorectal cancer, the lantern-shaped flexible origami can compress mRNA to nanoscale, thereby promoting its endocytosis by cells and improving translation efficiency. The mRNA nano-lantern facilitates the overexpression of Smad4, a tumor suppressor gene, in orthotopic colorectal tumor models, effectively inhibiting their growth [ 103 ]. This origami strategy offers a competitive DDS for mRNA-based therapies in the treatment of colorectal cancer. (4) Outer membrane vesicles (OMVs). OMVs contain numerous PAMPs that can effectively stimulate the innate immune system, facilitating T cell activation and antigen presentation. OMVs with surface decoration of lysosomal escape protein listeriolysin O and RNA binding protein, L7Ae, (OMV-LL) can be cross-presentation and OMV-LL mRNA significantly inhibits the progression of melanoma [ 104 ]. (5) Porous silica nanoparticles. This mRNA DDS is based on polyethylenimine-modified porous silica nanoparticles. It promotes effective antitumor immunity without evidence of systemic toxicity and off-target translation of mRNA [ 105 ]. It also inhibited distant metastatic tumors and improved anti-tumor responses in murine cancer models. (6) Iron oxide. This is a magnetically multi-functional RNA-loaded liposome that is capable of generating a robust anti-cancer immune response. Comparing to electroporation, this mRNA DDS activates DCs more effectively, resulting in superior tumor growth inhibition in animal models [ 106 ].
Deficiencies of the adjuvants of mRNA cancer vaccines
Current clinical trials of mRNA vaccines have shown that adverse reactions such as fatigue, pain at the injection site, myalgia, narcolepsy and neurological side effects can be triggered by the mRNA vaccines [ 107 , 108 , 109 ]. Notably, there are also a number of adverse reactions and limitations associated with adjuvants that need to be considered. Immune-related adverse event is one of the most notable side effects. Reports of anaphylactic reactions induced by mRNA vaccines are still being received [ 110 ]. At the injection site, adjuvanted vaccines are more reactogenic than non-adjuvanted vaccines, which can cause immune-related adverse events such as anaphylaxis. However, the symptoms are typically mild to moderate and of short duration [ 18 ]. Notably, immune activation and cytotoxicity may be triggered when injected above a certain dose of mRNA or ionizable lipids. Ultimately, allergic reactions and even cytokine storms may be triggered [ 111 ]. In preclinical mRNA vaccine studies, LNP were found to be highly inflammatory in mice, triggering a severe inflammatory response [ 112 ]. The ionizes lipids SM-102, which is ionizable and used in vaccines, may cause vaccinator to experience adverse effects such as nausea [ 113 ]. Besides, in PEGylated lipids, PEG may cause allergic reactions [ 114 ].
In addition, the unsatisfactory outcomes and unclear mechanisms of most approved adjuvants cannot be ignored. Comparatively, the mRNA vaccines have low immunogenicity and produce weak and short-lived immunity in the body [ 115 ]. The vaccine’s protective effect is low until two vaccinations were completed. Additionally, the effectiveness of vaccine declined with age, and is ineffective in the old age group [ 116 ]. Alternatively, the development of mRNA adjuvants is still in its early stages, and the construction of DDS for mRNA vaccines is immature. The mechanisms of many adjuvants remain unclear. Some basic problem such as the endosomal escape process remain unclear too [ 117 ]. Unclear mechanisms of adjuvants can result in improper use of adjuvants and the emergence of side effects. The deficiencies of the adjuvants of mRNA vaccines are summarized in Fig. 4 .
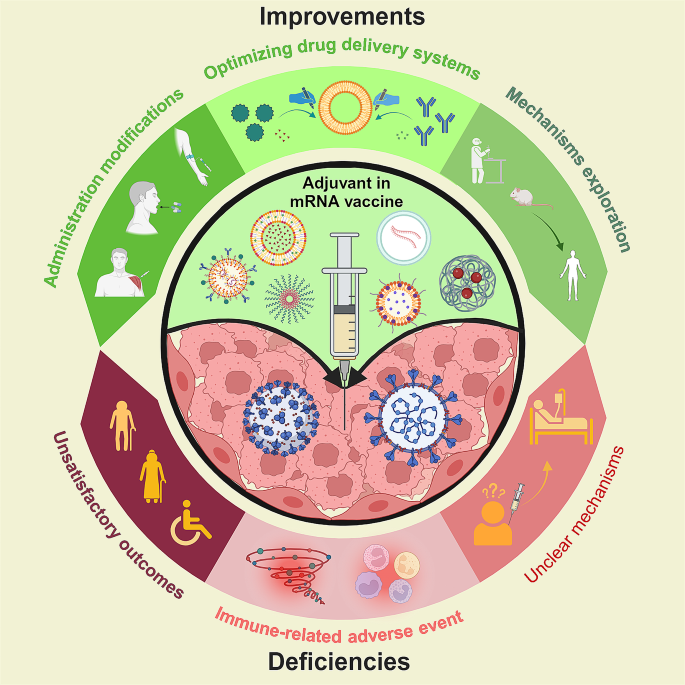
The deficiencies of mRNA adjuvants and the prospects for improvement. The immune-related adverse event, unsatisfactory outcomes in old age group and unclear mechanism of adjuvants are three main deficiencies of the mRNA adjuvants. Alternatively, optimizing drug delivery systems, improving route of administration and further explored the mechanisms of adjuvant are three main prospects for the improvement of mRNA adjuvants
Prospects for improvement
Further explored the mechanisms of adjuvant.
The formation of an immune niche (antigen depot), as mentioned above, is traditionally thought to be one of the important mechanisms of adjuvants. Nevertheless, with the deepening of researches, some researchers found that the removal of the “immune niche” after aluminum adjuvant administration didn’t substantial reduce the generation of B cell responses and antigen-specific T [ 118 ]. This suggests that the formation of an immune niche may not be the key mechanism of action of aluminum adjuvants. From this, we can further hypothesize whether other adjuvant systems are also like the aluminum adjuvant system. For more rational use of adjuvants and the development of new adjuvants, the mechanism of adjuvants should be better explored. Alternatively, the source of the adjuvant effect requires further researched. Immunostimulants and self-adjuvanted DDS for mRNA vaccines are not the only methods for achieving adjuvant effects. Editing the RNA itself can also produce adjuvant effect. However, the mechanism behind it requires further investigation [ 99 ].
Optimizing drug delivery systems
Using LNP as an example, DDS optimization can be achieved by optimizing lipid structure and targeted molecular. It has been shown that by modifying the lipid structure of lipid nanoparticles, including tail length, linkages and amine heads, and by optimizing the proportion of different lipid components of lipid nanoparticle formulations, lymph node-targeted delivery can be achieved to enhance vaccine immunity effects [ 77 , 119 ]. Notably, the modification of targeting molecules on the surface of LNP or alteration of the properties of the LNP can enhance the efficacy of vaccine by targeting LNP delivery to specific cells or organs. For example, mannosylation of lipid nanoparticles can enhance the uptake of APCs [ 120 ]. Modulation of the surface charge of RNA-lipid complexes enables precise and efficient targeting of DCs [ 121 ]. In addition, for non-liver tissues target, permanent cationic SORT lipids (EPC, DDAB, and DOTAP) can be applied to shift tissue tropism from the liver to the lung [ 13 ]. Alternating the alkyl length of a lipid or changing the intermediate connecting group of LNP from an ester bond to an amide bond in the tail can also change the organ targeting of LNP to liver or lung [ 122 , 123 ].
In addition to the existing DDS for mRNA vaccines, there are many novel DDS besides liposomes waiting to be discovered. For instance, engineered extracellular vesicles (EVs) with pathogen proteins is a promising alternative to LNP-mRNA vaccines. With their ability to naturally target and transport bioactive molecules, these engineered EVs are expected to overcome the problems of complex and non-continuous manufacturing processes and expensive materials involved in mRNA vaccine production [ 124 ].
Improving route of administration
Route of administration can greatly influence expression, kinetics organ distribution, and therapeutic outcome of LNP-mRNA vaccine [ 125 , 126 ]. Intravenous administration, as mentioned above, has the potential to enhance the immune response to mRNA vaccines, although issues of targeting non-liver tissues remain to be addressed [ 121 ]. Nevertheless, topical administration also has its own unique local therapeutic effect, allowing for the supplementation of therapeutic proteins in specific tissues such as brain, heart, eyes [ 127 , 128 , 129 ]. In addition, there are other routes of administration such as the intranasal route [ 130 ]. In order to better exploit the advantages of different routes of administration, a comprehensive decision on which route to use needs to be made after careful consideration of factors such as the nature of the nanoparticles and the therapeutic indications. The prospects for improving of mRNA vaccines are summarized in Fig. 4 .
Although there is still a long way to go in optimizing mRNA vaccines, their excellent biocompatibility, high tissue penetration, high nucleic acid encapsulation efficiency, low occurrence of off-target effects, cytotoxicity, and immunogenicity have made mRNA cancer vaccine one of the hottest research areas in vaccines today. Further researches are required to elucidate the mechanism of adjuvants and to optimize the design strategy and DDS for mRNA vaccines. Additionally, the development of new DDS for mRNA cancer vaccines and personalized mRNA cancer vaccines are promising avenues for future investigations. With the continued refinement of next-generation adjuvants, this new technology will help solve problems that traditional small-molecule and antibody therapies cannot, providing more effective and longer-lasting therapeutic outcome in the treatment of a wide range of diseases, including tumors, and improving healthcare in the near future.
Data availability
No datasets were generated or analysed during the current study.
Abbreviations
drug delivery systems
coronavirus disease 2019
antigen-presenting cells
dendritic cells
pathogen-associated molecular patterns
pattern recognition receptors
Toll-like receptors
myeloid differentiation primary response 88
nuclear factor kappa-light-chain-enhancer of activated B cells
extracellular signal-regulated kinase 1/2
interleukin-10
interleukin-12
lipopolysaccharide
monophosphoryl lipid
cytosine phospho-guanosine oligonucleotides
double-stranded DNA
mitochondrial DNA
guanosine monophosphate
adenosine monophosphate
cyclic GMP-AMP synthase
cyclic guanosine monophosphate-adenosine monophosphate
interferon regulatory factor 3
TANK-binding kinase
inhibitor of NF-kappaB kinase
cytotoxic T lymphocyte
C-type lectin receptors
nucleotide-binding oligomerization domain-like receptors
nucleotide-binding oligomerization domain 1
nucleotide-binding oligomerization structural domain 2
NOD-like receptor thermal protein domain associated protein 3
melanoma differentiation-associated gene 5
retinoic acid-induced gene I
interferon regulatory factor 7
interleukin-2
granulocyte-macrophage colony stimulating factor
interferon-alpha
tumor necrosis factor
α-galactosylceramide
poly(lactic-co-glycolic acid)
lipid nano-particle
B cell receptor
selective organ targeting
major histocompatibility complex II
major histocompatibility complex I
polyguanidine
RNA origami
outer membrane vesicles
OMVs with surface decoration of listeriolysin O and L7Ae
extracellular vesicles
Yang L, Gong L, Wang P, Zhao X, Zhao F, Zhang Z, Li Y, Huang W. Recent Advances in Lipid Nanoparticles for Delivery of mRNA. Pharmaceutics 2022, 14.
Baden LR, El Sahly HM, Essink B, Kotloff K, Frey S, Novak R, Diemert D, Spector SA, Rouphael N, Creech CB, et al. Efficacy and safety of the mRNA-1273 SARS-CoV-2 vaccine. N Engl J Med. 2021;384:403–16.
Article CAS PubMed Google Scholar
Whitley J, Zwolinski C, Denis C, Maughan M, Hayles L, Clarke D, Snare M, Liao H, Chiou S, Marmura T, et al. Development of mRNA manufacturing for vaccines and therapeutics: mRNA platform requirements and development of a scalable production process to support early phase clinical trials. Transl Res. 2022;242:38–55.
Lorentzen CL, Haanen JB, Met Ö, Svane IM. Clinical advances and ongoing trials on mRNA vaccines for cancer treatment. Lancet Oncol. 2022;23:e450–8.
Article CAS PubMed PubMed Central Google Scholar
Schlake T, Thess A, Thran M, Jordan I. mRNA as novel technology for passive immunotherapy. Cell Mol Life Sci. 2019;76:301–28.
Barbier AJ, Jiang AY, Zhang P, Wooster R, Anderson DG. The clinical progress of mRNA vaccines and immunotherapies. Nat Biotechnol. 2022;40:840–54.
Liu X, Huang P, Yang R, Deng H. mRNA Cancer vaccines: construction and boosting strategies. ACS Nano. 2023;17:19550–80.
Dunbar CE, High KA, Joung JK, Kohn DB, Ozawa K, Sadelain M. Gene therapy comes of age. Science 2018, 359.
Jinek M, Chylinski K, Fonfara I, Hauer M, Doudna JA, Charpentier E. A programmable dual-RNA-guided DNA endonuclease in adaptive bacterial immunity. Science. 2012;337:816–21.
Posey AD Jr., Schwab RD, Boesteanu AC, Steentoft C, Mandel U, Engels B, Stone JD, Madsen TD, Schreiber K, Haines KM, et al. Engineered CAR T cells targeting the Cancer-Associated Tn-Glycoform of the membrane mucin MUC1 control Adenocarcinoma. Immunity. 2016;44:1444–54.
Maruggi G, Zhang C, Li J, Ulmer JB, Yu D. mRNA as a transformative technology for Vaccine Development to Control Infectious diseases. Mol Ther. 2019;27:757–72.
Cobb M. Who discovered messenger RNA? Curr Biol. 2015;25:R526–532.
Palucka K, Banchereau J. Cancer immunotherapy via dendritic cells. Nat Rev Cancer. 2012;12:265–77.
Mellman I, Coukos G, Dranoff G. Cancer immunotherapy comes of age. Nature. 2011;480:480–9.
Rausch S, Schwentner C, Stenzl A, Bedke J. mRNA vaccine CV9103 and CV9104 for the treatment of prostate cancer. Hum Vaccin Immunother. 2014;10:3146–52.
Article PubMed PubMed Central Google Scholar
Rojas LA, Sethna Z, Soares KC, Olcese C, Pang N, Patterson E, Lihm J, Ceglia N, Guasp P, Chu A, et al. Personalized RNA neoantigen vaccines stimulate T cells in pancreatic cancer. Nature. 2023;618:144–50.
Mobasher M, Ansari R, Castejon AM, Barar J, Omidi Y. Advanced nanoscale delivery systems for mRNA-based vaccines. Biochim Biophys Acta Gen Subj. 2024;1868:130558.
Tavares Da Silva F, Di Pasquale A, Yarzabal JP, Garçon N. Safety assessment of adjuvanted vaccines: methodological considerations. Hum Vaccin Immunother. 2015;11:1814–24.
Article PubMed Google Scholar
Aldén M, Olofsson Falla F, Yang D, Barghouth M, Luan C, Rasmussen M, De Marinis Y. Intracellular reverse transcription of Pfizer BioNTech COVID-19 mRNA vaccine BNT162b2 in Vitro in Human Liver Cell line. Curr Issues Mol Biol. 2022;44:1115–26.
Zhao T, Cai Y, Jiang Y, He X, Wei Y, Yu Y, Tian X. Vaccine adjuvants: mechanisms and platforms. Signal Transduct Target Ther. 2023;8:283.
Li WA, Lu BY, Gu L, Choi Y, Kim J, Mooney DJ. The effect of surface modification of mesoporous silica micro-rod scaffold on immune cell activation and infiltration. Biomaterials. 2016;83:249–56.
Hafner AM, Corthésy B, Merkle HP. Particulate formulations for the delivery of poly(I:C) as vaccine adjuvant. Adv Drug Deliv Rev. 2013;65:1386–99.
Turley JL, Lavelle EC. Resolving adjuvant mode of action to enhance vaccine efficacy. Curr Opin Immunol. 2022;77:102229.
Luchner M, Reinke S, Milicic A. TLR Agonists as Vaccine Adjuvants Targeting Cancer and Infectious Diseases. Pharmaceutics 2021, 13.
Duthie MS, Windish HP, Fox CB, Reed SG. Use of defined TLR ligands as adjuvants within human vaccines. Immunol Rev. 2011;239:178–96.
Wang Y, Zhang S, Li H, Wang H, Zhang T, Hutchinson MR, Yin H, Wang X. Small-molecule modulators of toll-like receptors. Acc Chem Res. 2020;53:1046–55.
Ong GH, Lian BSX, Kawasaki T, Kawai T. Exploration of Pattern Recognition receptor agonists as candidate adjuvants. Front Cell Infect Microbiol. 2021;11:745016.
Maisonneuve C, Bertholet S, Philpott DJ, De Gregorio E. Unleashing the potential of NOD- and toll-like agonists as vaccine adjuvants. Proc Natl Acad Sci U S A. 2014;111:12294–9.
Watford WT, Moriguchi M, Morinobu A, O’Shea JJ. The biology of IL-12: coordinating innate and adaptive immune responses. Cytokine Growth Factor Rev. 2003;14:361–8.
Trinchieri G. Interleukin-12 and the regulation of innate resistance and adaptive immunity. Nat Rev Immunol. 2003;3:133–46.
Pulendran B. Modulating vaccine responses with dendritic cells and toll-like receptors. Immunol Rev. 2004;199:227–50.
Oberli MA, Reichmuth AM, Dorkin JR, Mitchell MJ, Fenton OS, Jaklenec A, Anderson DG, Langer R, Blankschtein D. Lipid nanoparticle assisted mRNA delivery for Potent Cancer Immunotherapy. Nano Lett. 2017;17:1326–35.
Mitchell TC, Casella CR. No pain no gain? Adjuvant effects of alum and monophosphoryl lipid A in pertussis and HPV vaccines. Curr Opin Immunol. 2017;47:17–25.
Zhuang X, Chen L, Yang S, Xia S, Xu Z, Zhang T, Zeng B, Yu T, Yu N, Wang W, et al. R848 Adjuvant Laden with Self-assembled nanoparticle-based mRNA vaccine elicits protective immunity against H5N1 in mice. Front Immunol. 2022;13:836274.
Barnett KC, Coronas-Serna JM, Zhou W, Ernandes MJ, Cao A, Kranzusch PJ, Kagan JC. Phosphoinositide interactions position cGAS at the plasma membrane to ensure efficient distinction between self- and viral DNA. Cell. 2019;176:1432–e14461411.
Wang Y, Luo J, Alu A, Han X, Wei Y, Wei X. cGAS-STING pathway in cancer biotherapy. Mol Cancer. 2020;19:136.
Diamond MS, Kinder M, Matsushita H, Mashayekhi M, Dunn GP, Archambault JM, Lee H, Arthur CD, White JM, Kalinke U, et al. Type I interferon is selectively required by dendritic cells for immune rejection of tumors. J Exp Med. 2011;208:1989–2003.
Van Herck S, Feng B, Tang L. Delivery of STING agonists for adjuvanting subunit vaccines. Adv Drug Deliv Rev. 2021;179:114020.
Lu T, Hu F, Yue H, Yang T, Ma G. The incorporation of cationic property and immunopotentiator in poly (lactic acid) microparticles promoted the immune response against chronic hepatitis B. J Control Release. 2020;321:576–88.
Liu Z, Zhou J, Xu W, Deng W, Wang Y, Wang M, Wang Q, Hsieh M, Dong J, Wang X, et al. A novel STING agonist-adjuvanted pan-sarbecovirus vaccine elicits potent and durable neutralizing antibody and T cell responses in mice, rabbits and NHPs. Cell Res. 2022;32:269–87.
Geijtenbeek TB, Gringhuis SI. Signalling through C-type lectin receptors: shaping immune responses. Nat Rev Immunol. 2009;9:465–79.
Lepenies B, Lee J, Sonkaria S. Targeting C-type lectin receptors with multivalent carbohydrate ligands. Adv Drug Deliv Rev. 2013;65:1271–81.
Engering A, Geijtenbeek TB, van Vliet SJ, Wijers M, van Liempt E, Demaurex N, Lanzavecchia A, Fransen J, Figdor CG, Piguet V, van Kooyk Y. The dendritic cell-specific adhesion receptor DC-SIGN internalizes antigen for presentation to T cells. J Immunol. 2002;168:2118–26.
Jiang W, Swiggard WJ, Heufler C, Peng M, Mirza A, Steinman RM, Nussenzweig MC. The receptor DEC-205 expressed by dendritic cells and thymic epithelial cells is involved in antigen processing. Nature. 1995;375:151–5.
Borriello F, Poli V, Shrock E, Spreafico R, Liu X, Pishesha N, Carpenet C, Chou J, Di Gioia M, McGrath ME, et al. An adjuvant strategy enabled by modulation of the physical properties of microbial ligands expands antigen immunogenicity. Cell. 2022;185:614–e629621.
Kvarnhammar AM, Petterson T, Cardell LO. NOD-like receptors and RIG-I-like receptors in human eosinophils: activation by NOD1 and NOD2 agonists. Immunology. 2011;134:314–25.
Rehwinkel J, Gack MU. RIG-I-like receptors: their regulation and roles in RNA sensing. Nat Rev Immunol. 2020;20:537–51.
Charerntantanakul W. Adjuvants for swine vaccines: mechanisms of actions and adjuvant effects. Vaccine. 2020;38:6659–81.
Ma X, Kadir Z, Li J, Zhang F. The effects of GM-CSF and IL-5 as molecular adjuvants on immune responses and contraception induced by mZP3 DNA vaccination. Am J Reprod Immunol. 2012;68:476–85.
Zeng Z, Wang H, Zhang Z, Yi Y. [Research progress of new vaccine adjuvants]. Sheng Wu Gong Cheng Xue Bao. 2021;37:78–87.
CAS PubMed Google Scholar
Kallen KJ, Heidenreich R, Schnee M, Petsch B, Schlake T, Thess A, Baumhof P, Scheel B, Koch SD, Fotin-Mleczek M. A novel, disruptive vaccination technology: self-adjuvanted RNActive(®) vaccines. Hum Vaccin Immunother. 2013;9:2263–76.
Weide B, Pascolo S, Scheel B, Derhovanessian E, Pflugfelder A, Eigentler TK, Pawelec G, Hoerr I, Rammensee HG, Garbe C. Direct injection of protamine-protected mRNA: results of a phase 1/2 vaccination trial in metastatic melanoma patients. J Immunother. 2009;32:498–507.
Kübler H, Scheel B, Gnad-Vogt U, Miller K, Schultze-Seemann W, Vom Dorp F, Parmiani G, Hampel C, Wedel S, Trojan L, et al. Self-adjuvanted mRNA vaccination in advanced prostate cancer patients: a first-in-man phase I/IIa study. J Immunother Cancer. 2015;3:26.
Papachristofilou A, Hipp MM, Klinkhardt U, Früh M, Sebastian M, Weiss C, Pless M, Cathomas R, Hilbe W, Pall G, et al. Phase ib evaluation of a self-adjuvanted protamine formulated mRNA-based active cancer immunotherapy, BI1361849 (CV9202), combined with local radiation treatment in patients with stage IV non-small cell lung cancer. J Immunother Cancer. 2019;7:38.
Zhang R, Tang L, Tian Y, Ji X, Hu Q, Zhou B, Zhenyu D, Heng X, Yang L. Cholesterol-modified DP7 enhances the effect of individualized cancer immunotherapy based on neoantigens. Biomaterials. 2020;241:119852.
Islam MA, Rice J, Reesor E, Zope H, Tao W, Lim M, Ding J, Chen Y, Aduluso D, Zetter BR, et al. Adjuvant-pulsed mRNA vaccine nanoparticle for immunoprophylactic and therapeutic tumor suppression in mice. Biomaterials. 2021;266:120431.
Verbeke R, Lentacker I, Breckpot K, Janssens J, Van Calenbergh S, De Smedt SC, Dewitte H. Broadening the message: a Nanovaccine co-loaded with Messenger RNA and α-GalCer induces Antitumor immunity through conventional and natural killer T cells. ACS Nano. 2019;13:1655–69.
Lehrman S. Virus treatment questioned after gene therapy death. Nature. 1999;401:517–8.
Hacein-Bey-Abina S, Von Kalle C, Schmidt M, McCormack MP, Wulffraat N, Leboulch P, Lim A, Osborne CS, Pawliuk R, Morillon E, et al. LMO2-associated clonal T cell proliferation in two patients after gene therapy for SCID-X1. Science. 2003;302:415–9.
Dirisala A, Uchida S, Li J, Van Guyse JFR, Hayashi K, Vummaleti SVC, Kaur S, Mochida Y, Fukushima S, Kataoka K. Effective mRNA protection by poly(l-ornithine) synergizes with endosomal escape functionality of a Charge-Conversion Polymer toward maximizing mRNA introduction efficiency. Macromol Rapid Commun. 2022;43:e2100754.
Patel S, Ashwanikumar N, Robinson E, DuRoss A, Sun C, Murphy-Benenato KE, Mihai C, Almarsson Ö, Sahay G. Boosting intracellular delivery of lipid nanoparticle-encapsulated mRNA. Nano Lett. 2017;17:5711–8.
Roth GA, Picece V, Ou BS, Luo W, Pulendran B, Appel EA. Designing spatial and temporal control of vaccine responses. Nat Rev Mater. 2022;7:174–95.
Adu-Berchie K, Mooney DJ. Biomaterials as local niches for Immunomodulation. Acc Chem Res. 2020;53:1749–60.
Jackson LA, Anderson EJ, Rouphael NG, Roberts PC, Makhene M, Coler RN, McCullough MP, Chappell JD, Denison MR, Stevens LJ, et al. An mRNA vaccine against SARS-CoV-2 - preliminary Report. N Engl J Med. 2020;383:1920–31.
Mulligan MJ, Lyke KE, Kitchin N, Absalon J, Gurtman A, Lockhart S, Neuzil K, Raabe V, Bailey R, Swanson KA, et al. Phase I/II study of COVID-19 RNA vaccine BNT162b1 in adults. Nature. 2020;586:589–93.
Wang YQ, Wu J, Fan QZ, Zhou M, Yue ZG, Ma GH, Su ZG. Novel vaccine delivery system induces robust humoral and cellular immune responses based on multiple mechanisms. Adv Healthc Mater. 2014;3:670–81.
Bachmann MF, Zinkernagel RM. Neutralizing antiviral B cell responses. Annu Rev Immunol. 1997;15:235–70.
Marcandalli J, Fiala B, Ols S, Perotti M, de van der Schueren W, Snijder J, Hodge E, Benhaim M, Ravichandran R, Carter L, et al. Induction of potent neutralizing antibody responses by a designed protein nanoparticle vaccine for respiratory Syncytial Virus. Cell. 2019;176:1420–e14311417.
Ingale J, Stano A, Guenaga J, Sharma SK, Nemazee D, Zwick MB, Wyatt RT. High-density array of Well-ordered HIV-1 spikes on synthetic liposomal nanoparticles efficiently activate B cells. Cell Rep. 2016;15:1986–99.
Soleimanpour S, Farsiani H, Mosavat A, Ghazvini K, Eydgahi MR, Sankian M, Sadeghian H, Meshkat Z, Rezaee SA. APC targeting enhances immunogenicity of a novel multistage Fc-fusion tuberculosis vaccine in mice. Appl Microbiol Biotechnol. 2015;99:10467–80.
Lu L, Palaniyandi S, Zeng R, Bai Y, Liu X, Wang Y, Pauza CD, Roopenian DC, Zhu X. A neonatal fc receptor-targeted mucosal vaccine strategy effectively induces HIV-1 antigen-specific immunity to genital infection. J Virol. 2011;85:10542–53.
Levin D, Golding B, Strome SE, Sauna ZE. Fc fusion as a platform technology: potential for modulating immunogenicity. Trends Biotechnol. 2015;33:27–34.
Shafifar M, Mozhgani SH, Razavi Pashabayg K, Mosavat A, Karbalaei M, Norouzi M, Rezaee SA. Selective APC-targeting of a novel Fc-fusion multi-immunodominant recombinant protein ((t)Tax-(t)env:mFcγ2a) for HTLV-1 vaccine development. Life Sci. 2022;308:120920.
Padera TP, Meijer EF, Munn LL. The lymphatic system in disease processes and Cancer Progression. Annu Rev Biomed Eng. 2016;18:125–58.
Kligerman J, Lima RA, Soares JR, Prado L, Dias FL, Freitas EQ, Olivatto LO. Supraomohyoid neck dissection in the treatment of T1/T2 squamous cell carcinoma of oral cavity. Am J Surg. 1994;168:391–4.
Bhagchandani S, Johnson JA, Irvine DJ. Evolution of toll-like receptor 7/8 agonist therapeutics and their delivery approaches: from antiviral formulations to vaccine adjuvants. Adv Drug Deliv Rev. 2021;175:113803.
Chen J, Ye Z, Huang C, Qiu M, Song D, Li Y, Xu Q. Lipid nanoparticle-mediated lymph node-targeting delivery of mRNA cancer vaccine elicits robust CD8(+) T cell response. Proc Natl Acad Sci U S A. 2022;119:e2207841119.
Kubara K, Yamazaki K, Miyazaki T, Kondo K, Kurotaki D, Tamura T, Suzuki Y. Lymph node macrophages drive innate immune responses to enhance the anti-tumor efficacy of mRNA vaccines. Mol Ther 2024.
Moyer TJ, Zmolek AC, Irvine DJ. Beyond antigens and adjuvants: formulating future vaccines. J Clin Invest. 2016;126:799–808.
Swartz MA. The physiology of the lymphatic system. Adv Drug Deliv Rev. 2001;50:3–20.
Jiang H, Wang Q, Sun X. Lymph node targeting strategies to improve vaccination efficacy. J Control Release. 2017;267:47–56.
Famta P, Shah S, Jain N, Srinivasarao DA, Murthy A, Ahmed T, Vambhurkar G, Shahrukh S, Singh SB, Srivastava S. Albumin-hitchhiking: fostering the pharmacokinetics and anticancer therapeutics. J Control Release. 2023;353:166–85.
Abdallah M, Müllertz OO, Styles IK, Mörsdorf A, Quinn JF, Whittaker MR, Trevaskis NL. Lymphatic targeting by albumin-hitchhiking: applications and optimisation. J Control Release. 2020;327:117–28.
Zhu G, Lynn GM, Jacobson O, Chen K, Liu Y, Zhang H, Ma Y, Zhang F, Tian R, Ni Q et al. Albumin/vaccine nanocomplexes that assemble in vivo for combination cancer immunotherapy. Nat Commun : 2017, 8:1954.
Dilliard SA, Cheng Q, Siegwart DJ. On the mechanism of tissue-specific mRNA delivery by selective organ targeting nanoparticles. Proc Natl Acad Sci U S A 2021, 118.
Hou X, Zaks T, Langer R, Dong Y. Lipid nanoparticles for mRNA delivery. Nat Rev Mater. 2021;6:1078–94.
Cruz FM, Colbert JD, Merino E, Kriegsman BA, Rock KL. The Biology and underlying mechanisms of Cross-presentation of Exogenous antigens on MHC-I molecules. Annu Rev Immunol. 2017;35:149–76.
Embgenbroich M, Burgdorf S. Current concepts of Antigen Cross-presentation. Front Immunol. 2018;9:1643.
Benjaminsen RV, Mattebjerg MA, Henriksen JR, Moghimi SM, Andresen TL. The possible proton sponge effect of polyethylenimine (PEI) does not include change in lysosomal pH. Mol Ther. 2013;21:149–57.
Du G, Sun X. Engineering nanoparticulate vaccines for enhancing antigen cross-presentation. Curr Opin Biotechnol. 2020;66:113–22.
Jerjes W, Theodossiou TA, Hirschberg H, Høgset A, Weyergang A, Selbo PK, Hamdoon Z, Hopper C, Berg K. Photochemical internalization for Intracellular Drug Delivery. From Basic mechanisms to Clinical Research. J Clin Med 2020, 9.
Otterhaug T, Janetzki S, Welters MJP, Håkerud M, Nedberg AG, Edwards VT, Boekestijn S, Loof NM, Selbo PK, Olivecrona H, et al. Photochemical internalization enhanced vaccination is safe, and gives Promising Cellular Immune responses to an HPV peptide-based vaccine in a phase I clinical study in healthy volunteers. Front Immunol. 2020;11:576756.
Haug M, Brede G, Håkerud M, Nedberg AG, Gederaas OA, Flo TH, Edwards VT, Selbo PK, Høgset A, Halaas Ø. Photochemical internalization of peptide antigens provides a Novel Strategy to realize therapeutic Cancer vaccination. Front Immunol. 2018;9:650.
Broz P, Dixit VM. Inflammasomes: mechanism of assembly, regulation and signalling. Nat Rev Immunol. 2016;16:407–20.
Zhu M, Du L, Zhao R, Wang HY, Zhao Y, Nie G, Wang RF. Cell-penetrating nanoparticles activate the Inflammasome to enhance antibody production by Targeting Microtubule-Associated protein 1-Light chain 3 for degradation. ACS Nano. 2020;14:3703–17.
Alameh MG, Tombácz I, Bettini E, Lederer K, Sittplangkoon C, Wilmore JR, Gaudette BT, Soliman OY, Pine M, Hicks P, et al. Lipid nanoparticles enhance the efficacy of mRNA and protein subunit vaccines by inducing robust T follicular helper cell and humoral responses. Immunity. 2021;54:2877–e28922877.
Qian J. mRNA vaccines: a powerful tool in cancer treatment. In International Conference on Biological Engineering and Medical Science . 2024.
Burris HA, Patel MR, Cho DC, Clarke JM, Gutierrez M, Zaks TZ, Frederick J, Hopson K, Mody K, Binanti-Berube A. A phase I multicenter study to assess the safety, tolerability, and immunogenicity of mRNA-4157 alone in patients with resected solid tumors and in combination with pembrolizumab in patients with unresectable solid tumors. American Society of Clinical Oncology; 2019.
Tockary TA, Abbasi S, Matsui-Masai M, Hayashi A, Yoshinaga N, Boonstra E, Wang Z, Fukushima S, Kataoka K, Uchida S. Comb-structured mRNA vaccine tethered with short double-stranded RNA adjuvants maximizes cellular immunity for cancer treatment. Proc Natl Acad Sci U S A. 2023;120:e2214320120.
Li W, Li Y, Li J, Meng J, Jiang Z, Yang C, Wen Y, Liu S, Cheng X, Mi S et al. All-trans-retinoic acid-adjuvanted mRNA vaccine induces mucosal Anti-tumor Immune responses for treating Colorectal Cancer. Adv Sci (Weinh) 2024:e2309770.
Zhang X, Wang K, Zhao Z, Shan X, Wang Y, Feng Z, Li B, Luo C, Chen X, Sun J. Self-Adjuvanting Polyguanidine Nanovaccines for Cancer Immunotherapy. ACS Nano. 2024;18:7136–47.
Yip T, Qi X, Yan H, Chang Y. RNA origami functions as a self-adjuvanted nanovaccine platform for Cancer Immunotherapy. ACS Nano. 2024;18:4056–67.
Hu M, Feng C, Yuan Q, Liu C, Ge B, Sun F, Zhu X. Lantern-shaped flexible RNA origami for Smad4 mRNA delivery and growth suppression of colorectal cancer. Nat Commun. 2023;14:1307.
Li Y, Ma X, Yue Y, Zhang K, Cheng K, Feng Q, Ma N, Liang J, Zhang T, Zhang L, et al. Rapid Surface Display of mRNA antigens by Bacteria-derived outer membrane vesicles for a personalized Tumor Vaccine. Adv Mater. 2022;34:e2109984.
Shin H, Kang S, Won C, Min DH. Enhanced local delivery of Engineered IL-2 mRNA by porous silica nanoparticles to promote effective Antitumor immunity. ACS Nano. 2023;17:17554–67.
Grippin AJ, Wummer B, Wildes T, Dyson K, Trivedi V, Yang C, Sebastian M, Mendez-Gomez HR, Padala S, Grubb M, et al. Dendritic cell-activating magnetic nanoparticles enable early prediction of Antitumor Response with magnetic resonance imaging. ACS Nano. 2019;13:13884–98.
Im JH, Kim E, Lee E, Seo Y, Lee Y, Jang Y, Yu S, Maeng Y, Park S, Park S, et al. Adverse events with the Pfizer-BioNTech COVID-19 vaccine among Korean Healthcare Workers. Yonsei Med J. 2021;62:1162–8.
Riad A, Pokorná A, Attia S, Klugarová J, Koščík M, Klugar M. Prevalence of COVID-19 Vaccine Side effects among Healthcare Workers in the Czech Republic. J Clin Med 2021, 10.
García-Grimshaw M, Ceballos-Liceaga SE, Hernández-Vanegas LE, Núñez I, Hernández-Valdivia N, Carrillo-García DA, Michel-Chávez A, Galnares-Olalde JA, Carbajal-Sandoval G, Del Mar Saniger-Alba M, et al. Neurologic adverse events among 704,003 first-dose recipients of the BNT162b2 mRNA COVID-19 vaccine in Mexico: a nationwide descriptive study. Clin Immunol. 2021;229:108786.
Banerji A, Wickner PG, Saff R, Stone CA Jr., Robinson LB, Long AA, Wolfson AR, Williams P, Khan DA, Phillips E, Blumenthal KG. mRNA vaccines to Prevent COVID-19 Disease and reported allergic reactions: current evidence and suggested Approach. J Allergy Clin Immunol Pract. 2021;9:1423–37.
Halamoda-Kenzaoui B, Bremer-Hoffmann S. Main trends of immune effects triggered by nanomedicines in preclinical studies. Int J Nanomed. 2018;13:5419–31.
Article CAS Google Scholar
Ndeupen S, Qin Z, Jacobsen S, Bouteau A, Estanbouli H, Igyártó BZ. The mRNA-LNP platform’s lipid nanoparticle component used in preclinical vaccine studies is highly inflammatory. iScience. 2021;24:103479.
Tahtinen S, Tong AJ, Himmels P, Oh J, Paler-Martinez A, Kim L, Wichner S, Oei Y, McCarron MJ, Freund EC, et al. IL-1 and IL-1ra are key regulators of the inflammatory response to RNA vaccines. Nat Immunol. 2022;23:532–42.
Kostoff RN, Calina D, Kanduc D, Briggs MB, Vlachoyiannopoulos P, Svistunov AA, Tsatsakis A. Retraction notice to Why are we vaccinating children against COVID-19? [Toxicol. Rep. 8 (2021) 1665–1684]. Toxicol Rep 2022, 9:1065.
Olotu A, Fegan G, Wambua J, Nyangweso G, Leach A, Lievens M, Kaslow DC, Njuguna P, Marsh K, Bejon P. Seven-year efficacy of RTS,S/AS01 malaria vaccine among young African children. N Engl J Med. 2016;374:2519–29.
Ranzani OT, Hitchings MDT, Dorion M, D’Agostini TL, de Paula RC, de Paula OFP, Villela EFM, Torres MSS, de Oliveira SB, Schulz W et al. Effectiveness of the CoronaVac vaccine in older adults during a gamma variant associated epidemic of covid-19 in Brazil: test negative case-control study. BMJ 2021, 374n2015.
Patel S, Kim J, Herrera M, Mukherjee A, Kabanov AV, Sahay G. Brief update on endocytosis of nanomedicines. Adv Drug Deliv Rev. 2019;144:90–111.
Hutchison S, Benson RA, Gibson VB, Pollock AH, Garside P, Brewer JM. Antigen Depot is not required for alum adjuvanticity. Faseb j. 2012;26:1272–9.
Zhang NN, Li XF, Deng YQ, Zhao H, Huang YJ, Yang G, Huang WJ, Gao P, Zhou C, Zhang RR, et al. A thermostable mRNA vaccine against COVID-19. Cell. 2020;182:1271–e12831216.
Zhuang X, Qi Y, Wang M, Yu N, Nan F, Zhang H, Tian M, Li C, Lu H, Jin N. mRNA vaccines encoding the HA protein of Influenza A H1N1 Virus delivered by Cationic lipid nanoparticles induce Protective Immune responses in mice. Vaccines (Basel) 2020, 8.
Kranz LM, Diken M, Haas H, Kreiter S, Loquai C, Reuter KC, Meng M, Fritz D, Vascotto F, Hefesha H, et al. Systemic RNA delivery to dendritic cells exploits antiviral defence for cancer immunotherapy. Nature. 2016;534:396–401.
Qiu M, Tang Y, Chen J, Muriph R, Ye Z, Huang C, Evans J, Henske EP, Xu Q. Lung-selective mRNA delivery of synthetic lipid nanoparticles for the treatment of pulmonary lymphangioleiomyomatosis. Proc Natl Acad Sci U S A 2022, 119.
Liu S, Cheng Q, Wei T, Yu X, Johnson LT, Farbiak L, Siegwart DJ. Membrane-destabilizing ionizable phospholipids for organ-selective mRNA delivery and CRISPR-Cas gene editing. Nat Mater. 2021;20:701–10.
Zhang B, Sim WK, Shen TL, Lim SK. Engineered EVs with pathogen proteins: promising vaccine alternatives to LNP-mRNA vaccines. J Biomed Sci. 2024;31:9.
Pardi N, Tuyishime S, Muramatsu H, Kariko K, Mui BL, Tam YK, Madden TD, Hope MJ, Weissman D. Expression kinetics of nucleoside-modified mRNA delivered in lipid nanoparticles to mice by various routes. J Control Release. 2015;217:345–51.
Melo M, Porter E, Zhang Y, Silva M, Li N, Dobosh B, Liguori A, Skog P, Landais E, Menis S, et al. Immunogenicity of RNA replicons encoding HIV Env Immunogens designed for self-assembly into nanoparticles. Mol Ther. 2019;27:2080–90.
Zangi L, Lui KO, von Gise A, Ma Q, Ebina W, Ptaszek LM, Später D, Xu H, Tabebordbar M, Gorbatov R, et al. Modified mRNA directs the fate of heart progenitor cells and induces vascular regeneration after myocardial infarction. Nat Biotechnol. 2013;31:898–907.
Nabhan JF, Wood KM, Rao VP, Morin J, Bhamidipaty S, LaBranche TP, Gooch RL, Bozal F, Bulawa CE, Guild BC. Intrathecal delivery of frataxin mRNA encapsulated in lipid nanoparticles to dorsal root ganglia as a potential therapeutic for Friedreich’s ataxia. Sci Rep. 2016;6:20019.
Patel S, Ryals RC, Weller KK, Pennesi ME, Sahay G. Lipid nanoparticles for delivery of messenger RNA to the back of the eye. J Control Release. 2019;303:91–100.
Kim J, Eygeris Y, Gupta M, Sahay G. Self-assembled mRNA vaccines. Adv Drug Deliv Rev. 2021;170:83–112.
Download references
Acknowledgements
Figures were created on BioRender.com.
This study was supported by the Fundamental Research Funds for the Central Universities (Wuhan University, Clinical Medicine + X, 2042024YXB017), Hubei Province Chinese Medicine Research Project (ZY2023Q015), Natural Science Foundation of Hubei Province (2023AFB665), Medical Young Talents Program of Hubei Province, Wuhan Young Medical Talents Training Project to L.-L. Bu., Youth Interdisciplinary Special Fund of Zhongnan Hospital of Wuhan University (ZNQNJC2022003), Health Commission of Hubei Province Scientific Research Project (WJ2023M068), Knowledge Innovation Program of Wuhan-Shugung Project (2023020201020510), Youth Fund of the National Natural Science Foundation of China (82001644), Medical Young Talents Program of Hubei Province (7020206), and “Dawn of Scientific and Technological Innovation” Program of Wuhan (703030804).
Author information
Lei-Ming Cao and Yi-Fu Yu contributed equally to this work.
Authors and Affiliations
State Key Laboratory of Oral & Maxillofacial Reconstruction and Regeneration, Key Laboratory of Oral Biomedicine Ministry of Education, Hubei Key Laboratory of Stomatology, School & Hospital of Stomatology, Wuhan University, Wuhan, 430079, China
Lei-Ming Cao, Yi-Fu Yu, Zi-Zhan Li, Nian-Nian Zhong, Guang-Rui Wang, Yao Xiao, Bing Liu & Lin-Lin Bu
Department of Oral & Maxillofacial - Head Neck Oncology, School & Hospital of Stomatology, Wuhan University, Wuhan, 430079, China
Bing Liu & Lin-Lin Bu
Department of Radiation and Medical Oncology, Hubei Key Laboratory of Tumor Biological Behavior, Hubei Provincial Clinical Research Center for Cancer, Zhongnan Hospital of Wuhan University, 169 Donghu Road, Wuhan, 430071, China
Department of Gynecology, Maternal and Child Health Hospital of Hubei Province, Tongii Medical College, Huazhong University of Science and Technology, Wuhan, China
You can also search for this author in PubMed Google Scholar
Contributions
C.L.: Methodology, Conceptualization, Writing - Original Draft, Writing - Review & Editing. Y.Y.: Investigation, Methodology, Conceptualization, Writing - Original Draft, Visualization, Writing - Review & Editing. L.Z.: Investigation, Methodology, Conceptualization, Writing - Original Draft, Writing – Review & Editing. Z.N.: Investigation, Methodology, Conceptualization, Writing - Original Draft, Writing – Review & Editing. W.G.: Investigation, Methodology, Conceptualization, Writing – Review & Editing. X.Y.: Writing - Review & Editing, Conceptualization. L.B.: Supervision, Writing - Review & Editing, Project Administration. W.Q.: Conceptualization, Methodology, Writing - Review & Editing, Supervision, Funding acquisition. F.C.: Supervision, Review & Editing, Funding acquisition. B.L.: Conceptualization, Supervision, Project Administration, Funding Acquisition, Writing - Review & Editing.
Corresponding authors
Correspondence to Qiu-Ji Wu , Chun Feng or Lin-Lin Bu .

Ethics declarations
Ethics approval and consent to participate.
Not applicable.
Consent for publication
Competing interests.
The authors declare no competing interests.
Additional information
Publisher’s note.
Springer Nature remains neutral with regard to jurisdictional claims in published maps and institutional affiliations.
Rights and permissions
Open Access This article is licensed under a Creative Commons Attribution 4.0 International License, which permits use, sharing, adaptation, distribution and reproduction in any medium or format, as long as you give appropriate credit to the original author(s) and the source, provide a link to the Creative Commons licence, and indicate if changes were made. The images or other third party material in this article are included in the article’s Creative Commons licence, unless indicated otherwise in a credit line to the material. If material is not included in the article’s Creative Commons licence and your intended use is not permitted by statutory regulation or exceeds the permitted use, you will need to obtain permission directly from the copyright holder. To view a copy of this licence, visit http://creativecommons.org/licenses/by/4.0/ . The Creative Commons Public Domain Dedication waiver ( http://creativecommons.org/publicdomain/zero/1.0/ ) applies to the data made available in this article, unless otherwise stated in a credit line to the data.
Reprints and permissions
About this article
Cite this article.
Cao, LM., Yu, YF., Li, ZZ. et al. Adjuvants for cancer mRNA vaccines in the era of nanotechnology: strategies, applications, and future directions. J Nanobiotechnol 22 , 308 (2024). https://doi.org/10.1186/s12951-024-02590-6
Download citation
Received : 13 April 2024
Accepted : 28 May 2024
Published : 02 June 2024
DOI : https://doi.org/10.1186/s12951-024-02590-6
Share this article
Anyone you share the following link with will be able to read this content:
Sorry, a shareable link is not currently available for this article.
Provided by the Springer Nature SharedIt content-sharing initiative
- mRNA cancer vaccine
- Drug delivery system
- Nanotechnology
Journal of Nanobiotechnology
ISSN: 1477-3155
- Submission enquiries: [email protected]
- Open access
- Published: 30 May 2024
The war between the immune system and the tumor - using immune biomarkers as tracers
- Kai Yang 1 na1 ,
- Rongrong Lu 1 na1 ,
- Jie Mei 1 na1 ,
- Kai Cao 1 ,
- Tianyu Zeng 1 ,
- Yijia Hua 1 , 2 ,
- Xiang Huang 1 ,
- Wei Li 1 &
- Yongmei Yin 1
Biomarker Research volume 12 , Article number: 51 ( 2024 ) Cite this article
156 Accesses
1 Altmetric
Metrics details
Nowadays, immunotherapy is one of the most promising anti-tumor therapeutic strategy. Specifically, immune-related targets can be used to predict the efficacy and side effects of immunotherapy and monitor the tumor immune response. In the past few decades, increasing numbers of novel immune biomarkers have been found to participate in certain links of the tumor immunity to contribute to the formation of immunosuppression and have entered clinical trials. Here, we systematically reviewed the oncogenesis and progression of cancer in the view of anti-tumor immunity, particularly in terms of tumor antigen expression (related to tumor immunogenicity) and tumor innate immunity to complement the cancer-immune cycle. From the perspective of integrated management of chronic cancer, we also appraised emerging factors affecting tumor immunity (including metabolic, microbial, and exercise-related markers). We finally summarized the clinical studies and applications based on immune biomarkers. Overall, immune biomarkers participate in promoting the development of more precise and individualized immunotherapy by predicting, monitoring, and regulating tumor immune response. Therefore, targeting immune biomarkers may lead to the development of innovative clinical applications.
As the third revolution in the history of drug therapy for malignant tumors, immunotherapy is regarded as one of the most promising anti-tumor therapies at present [ 1 ]. According to the immune surveillance theory, immunotherapy, which differs from conventional radiotherapy, chemotherapy, and targeted therapy, can leverage the anti-tumor immune system of patients to recognize and eliminate foreign tissues, such as tumors [ 2 ]. Additionally, it plays a crucial role in maintaining the equilibrium stage or potentially restoring the clear stage through reversing the escape stage, as per the tumor immunoediting theory, thereby effectively managing tumor progression over an extended period [ 3 ]. Due to its high universality, good effect on responders and low side effects, immunotherapy is no longer just a late-line treatment for patients with advanced tumors, but has gradually become the main means of tumor treatment, actively used in adjuvant or neoadjuvant therapy, and combined with other anti-tumor drugs such as targeting or anti-angiogenesis [ 4 ]. Due to the high side effects of chemotherapy drugs and low patient compliance, immunotherapy is gradually replacing chemotherapy drugs in front-line treatment in advanced lung cancer, leding to a new era of “chemo-free” treatment [ 5 ]. In addition, with the advancement of immunological knowledge and biological techniques, the emergence of “synthetic immunology” (primarily involving artificial manipulation and the creation of human immunity) has contributed to making immunotherapy more precise and effective [ 6 ].
However, there are still problems in immunotherapy: few patients respond to immunotherapy, and many of them have side effects mainly caused by drug resistance and immune-related adverse reactions (IRAEs). Meanwhile, the research of immunotherapy drugs is also mired in difficulties due to the lack of suitable targets [ 7 ]. In order to solve the above problems, we need to deeply study all aspects of immunogenesis and development of tumor to explore more suitable immune biomarkers as targets for monitoring and treatment.
Tumor immune biomarkers are biological features or indicators that can be objectively measured and used to evaluate tumor immune progression [ 8 ]. It is used to predict the efficacy and side effects of immunotherapy, monitor the immune response of tumors and provide targets for related drug research. Then we can screen out patients who are more suitable for immunotherapy, and may even make non-responders turn to responders or transform “cold tumors” to “hot tumors“ [ 9 ]. The treatment based on immune biomarkers breaks the concept of unified standardized treatment according to different tumor sources in the past, but carries out precise individual treatment according to the expression of markers [ 10 ]. In fact, various molecules (like receptors or cytokines), cells (of different types and proportions), microstructures (like tertiary lymphoid structures (TLS)), and even pathological features (like tumor cell death) involved in each stage of tumor immunity can be used as potential markers. However, attention should be paid to the extent of its contribution to the overall tumor immunity and the conversion possibility of clinical application [ 11 ]. Interestingly, in addition to naturally occurring ones during tumor immunity, biomarkers can also be artificially induced. For example, genetically engineered bacteria can specifically infect tumors while expressing corresponding antigens as biomarkers for immune cells to recognize tumors, and even produce substances similar to immune checkpoint inhibitors (ICIs) [ 12 ]. In addition to the exploration of the biomarkers themselves, the acquisition and detection means of immune biomarkers, the design of drug structure and the way of administration also affect the clinical application value of markers to a large extent [ 13 ].
Therefore, in order to search for immune biomarkers with high tumor specificity, good efficacy, and convenient clinical application, we updated and refined the rate-limiting aspects of the cancer-immunity cycle related to markers based on the remarkable progress of the past decade, particularly in terms of tumor antigen expression (related to tumor immunogenicity) and tumor innate immunity. The treatment of cancer is now gradually entering the “era of chronic disease management.” In addition to individualized treatment based on biomarkers, we also described comprehensive treatment, which includes the patient’s diet, exercise, and lifestyle habits. Finally, we focused on the clinical application and research progress based on tumor immune biomarkers.
Tumor immunity and its markers
The heterogeneity of tumor (primary drug resistance, Darwinian natural selection) and its ability to adapt to survival pressures (secondary drug resistance, Lamarkian evolution) result in the failure of therapeutic strategies that act directly on the tumor due to adaptive changes in the tumor target [ 14 ]. No matter how the tumor mutates, it is considered foreign to the body, exhibiting the behavior and characteristics of abnormal cells. Therefore, regulating the patients’ own tumor immune response sthrough immune biomarkers to indirectly fight against the tumor is a therapeutic strategy of “responding to all changes with no changes”. However, there are two main ways for the tumor to suppress anti-tumor immunity to form “cold tumors”. On the one hand, tumors can disguise themselves as normal cells to reduce their immunogenicity and thus reduce the detection of the immune system. On the other hand, tumors arm themselves with immunosuppressive tumor microenvironment (TME) to impede immune effects [ 15 ].
Initiation of tumor immune response
Tumor antigens are the basic biomarkers for the immune system to recognize tumors.
Tumor antigens are tumor biomarkers that are abnormally expressed with the development and progression of tumors, mainly due to abnormalities at the gene level, but also due to abnormalities in the antigen synthesis process, such as post-transcriptional RNA splicing disorder or post-translational protein modification disorder [ 16 ]. In addition, it takes time for mutations to accumulate in normal somatic cells until they affect genes crucial for cell proliferation and death, eventually leading to tumorigenesis. During this time, normal somatic cells can also produce tumor antigens due to mutations in tumor-related genes, and even induce anti-tumor immunity (similar to autoimmunity) in non-tumor situations [ 17 ].
On the one hand, when affected by external influences such as physical, chemical or biological carcinogenic factors, or by internal influences such as spontaneous DNA replication errors, the cell will have gene mutations, which belongs to classical genetics. It is mainly manifested as the imbalance between proto-oncogenes and tumor suppressor genes [ 18 ]. Changes in gene sequences cause tumor cells to produce antigens that normal cells do not express, namely tumor-specific antigens (TSAs, also known as neoantigens), with high immunogenicity and individual heterogeneity [ 19 ]. It is worth noting that the genetic mutations that cause carcinogenesis can be divided into a few key driver genes and a large number of accompanying passenger genes. Theoretically, the greater the number of mutations, that is, the higher the tumor mutation burden (TMB), the more TSAs will be produced, although not all mutations associated with TMB-H will enhance the antitumor immune response [ 20 ]. In addition, the quality of the mutation also affected the production of TSAs, The study found that TSAs mainly came from the mutation of non-driver genes, while the mutation of passenger genes has a weak effect on the fitness of tumor cells [ 21 ]. Mutations in the genome have been found to contribute to tumor growth in 5–10% of patients [ 22 ].
On the other hand, abnormal gene expression may occur in cells, It is mainly manifested in the differences in the timing, spatial distribution, and level of gene expression compared to normal physiological or non-cancerous pathological processes [ 23 ]. Because there is no genetic sequence change, tumor cells will produce antigens that normal cells can also express, that is, tumor-associated antigens (TAAs), which have weak immunogenicity due to the formation of immune tolerance. TAAs include cancerous testicular antigen, differentiated antigen, overexpressed antigen and cancerous embryo antigen [ 24 ]. Notably, some TAAs can also be derived from classical genetic pathways, such as gene recombination that reactivates silenced promoters. Unlike other TAAs, some carcinoembryonic antigens reexpressed later still have high immunogenicity because the expression of normal embryonic proteins precedes the formation of immune tolerance [ 25 ].
Different from TAA, TSA is more tumor specific and is key to initiating the body’s anti-tumor immunity and T cell effector activation [ 26 ]. However, tumor antigens are not only biomarkers of mediated immunity or anti-tumor drugs targeting tumors, some tumor antigens promote the occurrence and development of tumors to a large extent. For example, some breast cancer cells overexpress human epidermal growth factor receptor 2 (Her-2), which enhances cell proliferation and differentiation mediated by growth factor signaling [ 27 ]. Therefore, for tumors, the balance between the survival promotion effect of tumor antigens and the anti-tumor immunity they provoke determines the tumor immunophenotype, including sensitivity and resistance to immunotherapy. Overall, tumors tend to hide TSA and masquerade as normal cells by expressing TAA, mediating immune escape [ 28 ].
In addition to the antigens spontaneously produced in the above cellular carcinogenic pathways, foreign microbial antigens expressed by non-carcinogenic pathways, such as viruses and bacteria, can also be used as tumor antigens. Microbial antigens activate anti-microbial immunity while destroying host tumor cells [ 29 ]. According to the state of the infected cell, microbial antigens can be divided into two categories. The one type of antigens arise from the process in which normal cells get infected and transform into tumor cells. Most of them are only related to the early stage of tumor development, while the later stage of tumor progression is related to the carcinogenesis pathway it induces. Other normal cells will also produce the same antigens after being infected. The other type of antigens is expressed after specific infection of tumor cells, which are mostly expressed by genetically engineered bacteria [ 30 ]. In recent studies, human endogenous retroviruses (HERVs) have been found to be more than the remnants of ancient retroviruses with low transcriptional activity. They can be reactivated by several factors, such as environmental carcinogenic factors, which may drive the expression of oncogenes and activate anti-tumor innate immunity by inducing viral defense pathways [ 31 ].
Tumor cell death is a biomarker of the release of tumor internal antigens and the initiation of immune cell infiltration
Immune cells must be able to recognize and contact tumor antigens in order to initiate tumor immunity. The immune system isn’t able to actively detect tumors. For one thing, immune cells patrol and supervise the body’s cells, that is lymphocyte recirculation, to increase the chance of contact with tumor antigens. But the cells involved in this recirculation are mainly memory T and B cells, whose primary role is to maintain long-term immunity rather than to initiate initial immunity [ 32 ]. For another, tumor cells can hide tumor antigens by resisting senescence and death, reducing and modifying the expression of major histocompatibility complex (MHC), and gradually differentiate into less immunogenic phenotypes with the progress of tumor immunoediting [ 2 ].
However, the mismatch between uncontrolled tumor proliferation and local resource supply results in the lack of available resources and the accumulation of metabolic wastes, such as hypoxia, glucose deficiency, lactic acid accumulation and oxidative stress. It inevitably leads to tumor cell senescence, damage, such as endoplasmic reticulum (ER) stress, and non-apoptotic regulatory cell death (RCD), especially immunogenic cell death (ICD) [ 33 ]. The death of tumor cells will release tumor internal antigens, adenosine triphosphate (ATP) and high mobility group protein 1 (HMGB1) and other immune stimulators to activate the tumor immune response. Similarly, dying tumor cells can also express damage-associated molecular patterns (DAMPs), such as ER chaperone calreticulin (CRT/CALR) and heat shock protein (HSP), which attract and activate innate immune cells by binding to pattern recognition receptors (PRRs) [ 34 ]. In addition, senescent tumor cells also secrete a cocktail of proinflammatory cytokines to form the senescence-associated secretory phenotype (SASP), which in turn regulates TME. Changes in the proportion of senescent cells in tumors before and after treatment have been recognized as one of the hallmarks of cancer [ 35 ].
Therefore, the death or injury of tumor cells is an important biomarker for the release of tumor internal antigens and the initiation of immune invasion. It is often used as a clinical target to develop various therapeutic strategies for inducing non-apoptotic RCD in tumors, such as ICD inducers [ 36 ]. However, this does not explain why healthy tumor cells in early TME can be recognized by the immune system when resources are sufficient, especially before the immune system applies the selective pressure to screen for lower immunogenic phenotypes. In this regard, recent studies have found that differences in epigenetic modification of the RNA-binding protein cold shock domain-containing protein E1 (CSDE1) can lead to high or low immunogenic heterogeneity in early neonatal tumor cells [ 37 ].
However, the death or injury of tumor cells does not always initiate an anti-tumor immune response, and may even suppress tumor immunity. Since cell death often occurs inside the tumor tissue, it is possible that the ‘corpse’ of the tumor is buried by the TME and cannot be detected by immune cells [ 38 ]. Even if the immune infiltration is activated, most tumor cells can hide their own antigens and pretend to be ‘innocent bystanders’. At this time, a large number of infiltrated immune cells can act as ‘scavengers’ to dispose of the ‘corpse’, with the process of antigen presentation being inhibited by rapid removal of DAMPs and tumor debris, which induce immune tolerance [ 39 ]. It is worth noting that the ‘corpse’ of the tumor can also cause the ‘pollution’ of TME. For example, potassium ions released after tumor cell death can inhibit the antitumor effect of effector T cells (Teff) [ 40 ]. Released prostaglandin E2 (PGE2) can also inhibit the activation of DAMPs on macrophages and dendritic cells (DCs) [ 41 ]. Similarly, HMGB1 released after iron death of tumor cells induces polarization of M2-type macrophages by binding to AGE [ 42 ]. Besides, the high expression of CD39 and CD73 in TME can convert immune-activated ATP into immunosuppressive adenosine, thus forming a negative feedback mechanism of adenosine energy axis conducive to tumor development [ 43 ]. On the contrary, the death of the; siblings’ can cause other tumor cells to be alert and enter a ‘state of combat readiness’. Similar to innate immune cells, tumor cells can also express PRRs such as toll-like receptors (TLRs) and P2 × 4 purinergic receptors to recognize DAMPs molecules such as ATP. Then they can activate signaling pathways such as NF-κB and mitogen-activated protein kinase (MAPK), which promote tumor cell proliferation and resist death. What’s more, it induces the production of various inflammatory factors and chemokines to regulate the differentiation and recruitment of immune cells [ 44 ].
Biomarkers of tumor innate immunity
The role of innate immunity can be roughly divided into two categories. One is to attack tumor cells directly through phagocytosis, including natural killer cells (NK cells) and macrophages. The other group activates the second line of defense against tumors, namely adaptive immunity, mainly involving DCs through antigen presentation [ 45 ]. In order to counter the innate immune response, tumor cells can directly affect the camp of innate immune cells, the bone marrow, by inhibiting the maturation of DCs, granulocytes, and macrophages to eventually form and recruit immunosuppressive myeloid-derived suppressor cells (MDSCs). MDSCs can be classified as granulocyte/polymorphonuclear cells MDSCs (PMN-MDSCs, CD11b + CD14-CD15+/CD66b+) and monocyte MDSCs (M-MDSCs, CD14 + CD15-HLA-DRlo/-) [ 46 ]. It is worth noting that the recent study has also found tumors can induce CD45 + erythroid progenitor cells (EPCs) to transdifferentiate into erythroid derived myeloid cells (EDMCs), thereby supplementing MDSCs at the source [ 47 ].
Build the first line of defense against tumor
NK cells are native cells with surface markers CD3-CD19-CD56 + CD16+ (CD3 is a marker for T cells and CD19 is a marker for B cells) and intracellular transcription factor E4BP4+. According to the difference of CD56 expression, NK cells can be divided into CD56dim, which mainly plays a cytotoxic role, and CD56bright, which mainly plays an immunomodulatory role [ 48 ]. Like T cells, subtypes such as regulatory NK cells, NK cell depletion, and tissue-resident NK cells have been identified [ 49 ]. Given the significant heterogeneity of NK cell infiltration in tumors, NK cell frequency predicts the efficacy of immunotherapies such as anti-programmed death receptor-1 (PD-1) [ 50 ]. Unlike Teff, NK cells do not express specific antigen-recognition receptors, but regulate activation state primarily through the balance of a series of activated killer cell receptors (AKRs) and inhibitory killer cell receptors (IKRs) signals (Figs. 1 and 2 ). NK cells do not require antigen presentation and have a broader spectrum, rapid, and safe (for allotransplantation) antitumor effect [ 51 ]. Therefore, AKRs and IKRs can be used as NK cell-associated immune checkpoint therapy (ICT) markers.
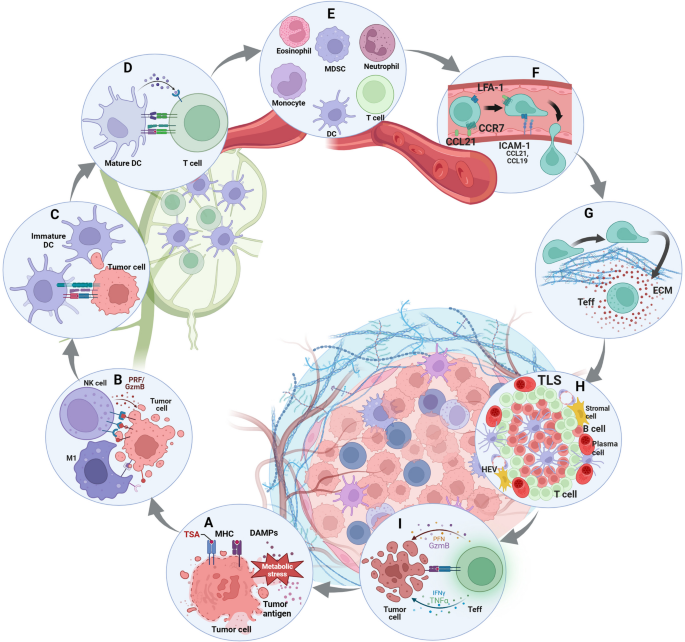
Various links in the development of tumor immunity. Initiation of the tumor immune response: A induce tumor cell ICD to release tumor antigens or promote tumor cell surface expression of pMHC to enhance immunogenicity. Building the first line of defense against tumors: B antitumor innate immune responses, including NK cells and macrophages, kill tumor cells through a balance of inhibitory and activating signals. Activated the second line of anti-tumor defense: C DC captured tumor antigens and migrated to TDLN and gradually matured. D DCs present antigen to T cells. The march of immune cells into the tumor stronghold: E chemokine-regulated immune recruitment in the peripheral blood circulation. F permeable malformed tumor neovascularization. G penetrate the tumor ECM with a rigid structure and immunosuppressive properties. Immune cells form the provisional command of the front line: H the formation of TLS. T cells exert effect: I T cells kill tumor cells through a balance of three signal s
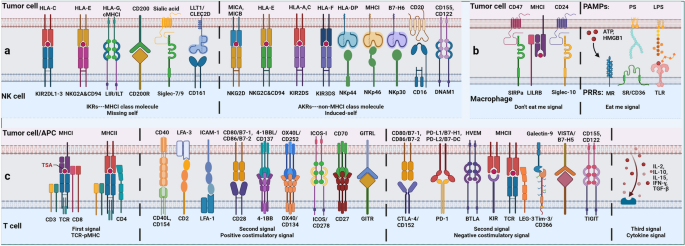
Balance between inhibitory and activation of cell-surface immune checkpoints. Anti-tumor innate immunity: NK cells regulate their activation state mainly through the balance of a series of activating killer cell receptors (AKRs, mainly recognizing non-MHCI molecules) and inhibitory killer cell receptors (IKRs, mainly recognizing MHCI molecules). Macrophages regulate their activation state mainly through the balance of a series of ‘eat me’ (DAMPs) and ‘don’t eat me’ (SIRPa) signals. Anti-tumor adaptive immunity: T cells regulate their activation state mainly through the balance of a series of TCR-pMHC, costimulatory molecules and cytokine signaling
In general, non-MHCI molecules on the tumor surface activate NK cells after binding with AKRs while MHCI molecules suppress NK cells after binding with IKRs, but MHCI molecules can also activate AKRs [ 52 ]. In order to avoid the recognition of Teff, tumor cells usually down-regulate the expression of MHCI or have gene mutations of human leukocyte antigen (HLA) and β2 microglobulin (β2m). The loss of MHCI will mediate the killing of NK cells, that is, the ‘missing self’ effect, but that process can be disrupted. Due to the influence of TME, the number of NK cells infiltrated in the tumor was less, especially the subgroups with cytotoxic effects [ 53 ]. In addition, tumor cells can regulate the balance of AKRs and IKRs to keep NK cells in an inhibited state. For example, the non-classical HLA gene HLA-E has been shown to be highly expressed in various tumors and can provide inhibitory signals to NK cells and CD8 + αβT cells expressing NKG2A/CD94 [ 54 ].
Macrophages in tumors are mainly derived from circulating monocytes and tissue-resident macrophages, both of which form tumor-associated macrophages (TAMs) under the influence of TME [ 55 ]. In the early stage of immune response, like pro-inflammatory M1 type, TAM mainly plays a killing role, with high expression of CD80, CD86, MHCII, inducible nitric-oxide synthase (iNOS) and CD68 and is dependent on glycolysis. In the later stage of immune response, like anti-inflammatory M2 type, TAM mainly plays an immunosuppressive role, highly expressing CD206, CD204, vascular endothelial growth factor (VEGF), CD163 and arginase (Arg-1), and relying on fatty acid oxidation (FAO) [ 56 ]. The study has shown that cell frequency of TAMs is related to response to tumor immunotherapy [ 57 ]. Similar to NK cells, TAM regulates activation state mainly through the balance of a series of ‘eat me’ and ‘don’t eat me’ signals which act as a macrophage-related ICT marker. For example, DAMPs expressed by tumor cells convey a ‘eat me’ signal to macrophages, but MHCI expressed by tumor cells conveys a ‘don’t eat me’ signal [ 58 ].
Activate the second line of anti-tumor defense
Only DCs can activate initial T cells (Th0) in tumor draining lymph nodes (TDLNs) to initiate an adaptive immune response de novo [ 59 ]. Tumor of immature DCs have high expression of PRRs but low expression of MHC, co-stimulatory and adhesion molecules so that they have strong antigen uptake and processing ability but weak ability to present antigen. In the process of migration to TDLN, DCs gradually mature and exhibit receptor expression and function opposite to the immature state [ 60 ]. Notably, tumors inhibit the maturation of DC through immunosuppressive TME, specifically tumor extracellular matrix (ECM) related components. For example, the presentation of tumor antigens can be inhibited by mechanisms such as DC-dependent T cell activation induced cell death (AICD) [ 61 ]. The maturation of type 1 conventional dendritic cells (cDC1) was found to be interfered with by NF-κB and specific inactivation of interferon regulatory factor 1 (IRF1) [ 62 ]. More importantly, tumor-derived immunosuppressive factors (such as transforming growth factor β (TGF-β)) and tumor cells with lymph node metastasis can reshape the TDLN microenvironment and directly affect the production of Teff [ 63 ].
Unlike the state of individual cell types, the collection of cells with functional connections, that is, the immune archetype, better reflects the intrinsic connections and interactions of tumor immunity [ 64 ]. For example, cDC1 mainly supports the effects of proliferative tumor-antigen specific TCF-1 + CD8 + T cells and is regulated by chemokines secreted by NK cells [ 65 ]. Type 2 conventional dendritic cell (cDC2) mainly supports the effects of CD4 + T cells and is inhibited by their derivative subtype regulatory T cells (Tregs) [ 66 ]. Contrary to conventional wisdom, the recent study has shown that cDC1 can activate CD4 + T cells through MHCII cross-presentation of tumor antigens. In turn, CD4 + T cells can also promote cDC1 function through CD40 signaling [ 60 ]. In addition, a subpopulation of DCs characterized by immunomodulatory genes, namely mature regulatory dendritic cell (mregDC) rich in immunomodulatory molecules, are associated with uptake of dead tumor cells and inhibit the role of cDC1-activated T cells in TDLN [ 67 ]. Therefore, all the molecules, cell types and TDLN microenvironment involved in the antigen presentation process of DCs and T cells can be used as biomarkers of immunotherapy.
Biomarkers of tumor adaptive immunity
The strategies of innate and adaptive immunity to recognize tumors are different. Innate immunity mainly recognizes the absence of normal cell biomarkers through the ‘missing self’ effect while adaptive immunity mainly recognizes the appearance of abnormal tumor biomarkers by binding T cell receptors (TCRs) to antigenic peptide-MHC (pMHC) [ 68 ]. Among them, tumor adaptive immunity mainly relies on T cells. But for tumor humoral immunity, on the one hand, tumor-specific antibodies can activate complement-dependent cytotoxicity (CDC), antibody-dependent cell-mediated cytotoxicity (ADCC), and antibody-dependent cell-mediated phagocytosis (ADCP) of NK cells and macrophages, and even block some tumor-promoting growth factor receptors [ 69 ]. On the other hand, tumor-specific antibodies cannot target internal tumor antigens when the expression level of tumor surface antigens is low [ 70 ]. Certain boosting antibodies or tumor-targeting monoclonal antibodies (mAbs) can even inhibit the cytotoxic effect of NK and T cells by promoting the expression of PD-L1 and indoleamine 2, 3-dioxygenase (IDO). They can also promote tumor metastasis by binding to cell adhesion molecules [ 71 ].
Immune cells enter the tumor base camp
Although current immunotherapy mainly focuses on remodeling the local TME, more and more studies have begun to focus on the systemic immunity beyond tumors, including TDLN, bone marrow, and peripheral blood circulation. The study has found that the frequency of immune cells and the level of immune molecules in blood circulation can be used as potential markers for immunotherapy [ 72 ]. In addition, Teff in TME in response to ICT are mainly derived from the peripheral input of T cell precursors of exhaustion (Tpex) in TDLN rather than from local T cell exhaustion (Tex), indicating that immunotherapy not only regulates the local TME, but also changes the systemic immunity of whole body [ 73 ].
With the function of the corresponding chemokines, immune cells are released from the TNDL and bone marrow into the bloodstream and directed to the site of the tumor immune response. But chemokine secretion in TME is often altered [ 74 ]. Since different immune cell subpopulation has different chemokine receptor expression patterns, abnormal expression of chemokines may regulate the differentiation, recruitment, homing and recycling of lymphocytes according to the needs of tumor survival or therapeutic intervention [ 75 ]. In addition, chemokines are involved in tumor angiogenesis, proliferation, and invasiveness by directly targeting non-immune cells in TME, such as tumor, stromal, and vascular endothelial cells. Therefore, chemokines are important biomarkers of tumor immunity and valuable therapeutic targets [ 76 ].
However, the process by which immune cells infiltrate tumor is hindered in many ways. The first barrier that immune cells encounter in the circulation of the blood is the endovascular glycocalyx formed by the ECM. The malformed tumor neovascularization system prevents the immune cells from entering the tumor [ 77 ]. Depletion of the Rgs gene has been found to normalize tumor vasculature, thereby promoting immune cell infiltration [ 78 ]. Different from the classical endothelium-dependent tumor angiogenesis pathway, tumor vasculogenic mimicry (VM) is a novel tumor microcirculation model independent of the body’s endothelial cells. The vascular pathway is constructed by the tumor cells themselves. Although there is a lack of direct studies to prove the effect of VM on tumor immunity, it can be speculated that the absence of endothelial cells impedes the endothelium-dependent adhesion and migration of immune cells, thereby hindering immune infiltration and distribution within the tumor. More importantly, rigid structure and immunosuppressive tumor ECM, especially collagen secreted by cancer-associated fibroblasts (CAFs), not only hinder the infiltration of immune cells. They also reduce the release of tumor antigens, interfere with antigen presentation and affect the effect of T cells, and even prevent the penetration of anti-tumor drugs [ 79 ]. However, ECM, as a protective shell of the tumor, can also hinder the outward progression and metastasis of the tumor, so the dissolution of ECM is one of the signs of tumor metastasis [ 80 ].
Immune cells form a temporary command line
In order to combat the immunosuppressive TME, immune cells in tumors do not fight alone but form a systematic team battle and intratumoral immunity cycle. This is evident in the formation of a densely populated area with MHCII high expression APC and CD8 + T cells, known as the APC niche, based on the immune archetype [ 81 ]. They may also form more types of lymphoid structures that regularly gather immune cells, known as TLS. The APC niche and TLS not only reflect the number and location of immune cell infiltration in the tumor, but also reflect the heterogeneity of its spatial distribution and the interaction between functions [ 82 ]. The study has found that the APC niche is associated with producing an effective and long-lasting immunotherapy response, but it is unclear whether the APC niche is an early stage of TLS. Similarly, the study has found that tumor-specific CD8 + T cells aggregated in TLS are rarely functionally exhausted and exhibit typical memory characteristics [ 83 ]. The study has also found that TLS mediates B cell maturation, plasma cell differentiation, and antibody formation [ 84 ]. Therefore, the development of TLS is associated with improved ICT efficacy, which can enhance immune infiltration and effect, and generate anti-tumor immune sites [ 81 ]. . Therefore, the APC niche and TLS are important markers for immunotherapy. However, TLS is also affected by tumor-produced cytokines and metabolic factors, such as TLS-associated Tregs, regulatory B cells (Bregs), interleukin-10 (IL-10), and enhanced antibodies, which lead to the formation of immunosuppressive TLS and macrophage or NK-cell-dependent apoptosis [ 85 ].
Process and outcome of T cell effect
Diffrent from the classical mode of activation for acute bacterial or viral infections, T cell activation of tumor can be divided into two stages: initial activation of cDC antigen presentation in TDLN and subsequent effector activation within the tumor [ 86 ]. Among them, effector activation can be roughly divided into three signals: TCR-pMHC, co-stimulatory molecules and cytokines [ 86 ]. Interestingly, the effect of T cells is not limited to single one cell, but is accumulated with the number of cells and the duration of action, that is, additive cytotoxicity. Particularly in solid tumors, sublethal injury events delivered by multiple T cells to a single tumor cell mediate effective tumor killing through time-dependent integration [ 87 ].
Most current studies focus on CD8 + T cells and tumor-constitutionally expressed MHCI, but the cytotoxic effects of CD4 + T cells and tumor-induced expression of MHCII also deserve our attention. The study has shown that interferon-γ (IFN-γ) induces tumor cells to express MHCII through MHC class II transactivator (CIITA), which is recognized and killed by cytotoxic CD4 + T lymphocyte (CD4 + CTL) [ 88 ]. Among them, class I MHC-restricted T-cell-associated molecules (CRTAM) can be used as early biomarkers of CD4 + CTL [ 89 ]. The effects of CD4 + CTL require external stimulation to be maintained [ 90 ]. Unlike MHCI, MHCII can bind to a higher diversity of antigenic peptides, thereby increasing the likelihood of CD4 + CTL recognition of TSA [ 88 ]. More importantly, the study found that MHCI and MHCII are independently regulated in tumor immunity, so their expression may have independent implications for immunotherapy [ 91 ].
Tumor cells can evade T-cells’ killing function in a variety of ways. In TDLN, immature DCs, upregulated TGF-β and down-regulated IL-2 can maintain T cells in quiescence. The transcription factor FOXO1 and its induced Kruppel-like factor 2 (KLF2) as well as V-domain Ig suppressor of T cell activation (VISTA) were highly expressed in static T cells [ 92 ]. However, tumor cells downregulate the expression of MHCI to induce the loss of TCR-pMHC signal, thus keeping T cells ignorant. Unlike quiescence, ignoring T cells can not specifically recognize tumor antigens [ 93 ]. However, even if the TCR-pMHC signal can be activated, the tumor can still maintain T cell anergy by downregulating positive co-stimulatory molecules [ 94 ]. The expression of IL-12, IFN-γ and tumor necrosis factor (TNF) was significantly decreased by anergic T cells. Only in the absence of tumor antigen stimulation can anergic T cells gradually resume their functional response [ 95 ]. However, interestingly, even in the absence of external activation from tumor cells, T cells can activate CD28 costimulatory signals by cis-B7:CD28 interactions at invaginated synapticmembranes, that is autologous signaling, thereby enhancing their ability to attack tumors [ 96 ].
In addition to down-regulating positive costimulatory molecules, tumor cells can also up-regulate the expression of negative costimulatory molecules. This helps maintain T cells in a state of depletion under the influence of stimulatory factors in TME such as chronic TCR signaling [ 97 ]. Genetic analysis revealed extensive chromatin remodeling and a close correlation with transcriptional regulatory factor TOX in Tex [ 98 ]. Although Tex overlaps with anergic T cells in the expression of inhibitory receptors, anergic T cells appear primarily early in immune response while Tex is produced late from Teff [ 99 ]. Based on the expression of Ly108 (representing transcription factor TCF1) and CD69, Tex is divided into four stages, namely, T cell exhaustion progenitors 1 (TexProg1), T cell exhaustion progenitors 2 (TexProg2), T cell exhaustion intermediate (TexInt) and T cell exhaustion terminally (TexTerm). Starting from TexInt, Tex gradually loses the expression of TCF1, so anti-PD-1 treatment can restore the function of Tex in the first three stages, which may be related to the fact that Tex has not completed the secondary epigenetic regulation [ 100 ]. However, not all T cells will be exhausted and lose function. The study has found that some T cells can be pluripotent and regenerative after depletion, that is Tpex. Unlike Tex, Tpex can express CD62L, TCF1, ID3, CXCR5, Ly108 and the transcription factor MYB [ 101 ]. Tpex is essential to maintain the Tex library and facilitate the response of ICT. Further study showed that cDC1 insulates Tpex from persistent tumor antigens through the action of pMHCI-TCR to prevent its further depletion [ 102 ]. TGF-β has also been shown to be closely related to the immune signal of depletion [ 103 ].
In addition, activated T cells can also be induced to develop AICD (peripheral deletional tolerance/death) in the absence of growth factors (such as IL-2) by apoptosis factors such as FasL, TNF and TNF-related apoptosis-inducing ligand (TRAIL) in TME [ 104 ]. It was found that the mutual inhibition of long non-coding RNA (lncRNA) NKILA and nuclear foctor-κB (NF-κB) can regulate the sensitivity of T cells to AICD [ 105 ]. In conclusion, multi-dimensional integrated T cell profiling, including TCR antigen specificity, sequence analysis, T cell phenotype, activation/exhaustion state, and clonal proliferation, is essential for the monitoring of T cell-based cancer immunotherapy [ 106 ].
Emerging important factors affecting tumor immunity
Competition for resource supply: metabolism-related biomarkers.
Tumor and immune cells adapt to various stress challenges in TME, such as hypoxia, nutritional competition (deficiency of glucose and key amino acids), acidic environment, and oxidative stress (lipid peroxidation), by changing their own metabolic patterns, that is, metabolic reprogramming [ 107 ]. The enhancement of aerobic glycolysis is an important sign of T cell activation and anti-tumor effect. But tumor cells rely on anaerobic glycolysis, that is “Warburg effect”, leading to cell competition for glucose [ 108 ]. In addition, tumors of non-gluconeogenic tissues, except for liver and kidney cancer, are able to compensate for glucose deficiency through partial activation of the gluconeogenic pathway [ 109 ]. In addition to being an alternative energy source, enhanced lipid metabolism promotes tumor invasion and metastasis, which is usually manifested as an increase in the proportion of unsaturated fatty acids (especially monounsaturated fatty acids) on the cell membrane and the accumulation of intracellular cholesterol. However, lipid metabolism promotes the transformation of immune cells to a protumor phenotype and inhibits the function of anti-tumor immune cells [ 110 ]. Similarly, tumor cells and immune cells compete for key amino acids, such as glutamine (Gln), which is essential for cell proliferation, metabolite production, and fatty acid synthesis [ 111 ]. In addition, the overexpression of IDO in tumor cells promotes the essential amino acid tryptophan (Trp) to catalyze kynurenine (Kyn). It leads to both the suppression of anti-tumor T cells caused by Trp deficiency and the activation of tumor-promoting immune cells mediated by Kyn accumulation [ 112 ]. Notably, some metabolism-regulating drugs can be used as concomitant drugs to promote tumor immunotherapy. For example, statins inhibit their ability to stabilize the expression of PD-1/PD-L1 on the cell membrane by blocking the synthesis of cholesterol [ 113 ]. In addition, metformin promoted the transformation of TME to an antitumor phenotype by increasing the number of CD8 + T cells while decreasing the expression of M2 macrophages (Fig. 3 ) [ 114 ].
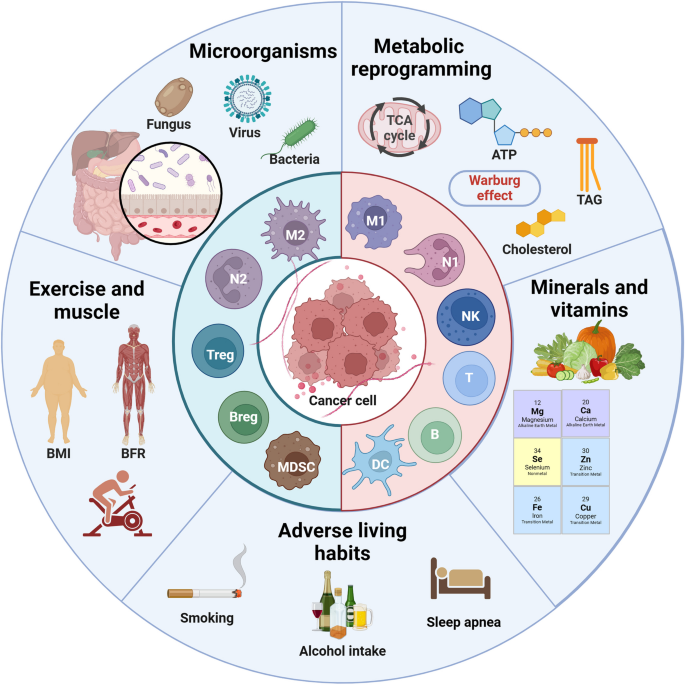
Important off-field factors affecting tumor immunity. In addition to tumor immunity itself, metabolic reprogramming (especially lipid metabolism) of tumor and related immune cells, as well as specific trace elements and vitamins affect the energy competition between cells at the metabolic level. As a new field of tumor immunity, tumor microbes and the microenvironment they create affect various aspects of tumor immunity, such as tumor immunogenicity, antigen presentation, ICD, metabolism, and ICs. In addition, exercise and bad habits also play an auxiliary role in tumor immune response that cannot be ignored. The above factors can be used as potential targets for regulating TME and improving immunotherapy
Supply of resource supplements: trace element and vitamin related biomarkers
Nutritional status is closely related to immune homeostasis, especially for cancer which is a wasting disease. The changes in the levels of related trace elements and vitamins in the body will affect the tumor immune response [ 115 ]. Carcinogenic trace elements are mostly heavy metal elements, such as arsenic, chromium, nickel, beryllium, cadmium and lead, while anti-cancer trace elements, such as selenium, magnesium, zinc, molybdenum, iron, calcium and copper. For example, selenium compounds protect cell-membrane structures and promote antitumor immune responses, primarily through antioxidant effects, particularly the clearance of lipid peroxides [ 116 ]. Similarly, Ca² + , Fe² + and Cu² + also affect the REDOX balance and mediate Ca² + overload, ferroptosis and cuproptosis of tumor cells to activate anti-tumor immune responses [ 110 ]. In addition, zinc also promotes lipid metabolism and decreases cellular lipid supply and accumulation [ 117 ]. It is worth noting that trace elements have a relatively strict proportion in the body. High or low content or improper proportion of each element will affect tumor immunity. For example, excessive iron will also lead to oxidative stress of immune cells, resulting in ferroptosis and inhibition of immune function [ 118 ]. In addition to trace elements, vitamins also affect tumor immunity. For example, high-dose vitamin C can increase T-cell infiltration and improve the efficacy of ICT [ 119 ]. Similarly, dietary supplementation with vitamin E could enhance patient response to ICT by increasing tumor antigen presentation and activating T cell-mediated antitumor effects [ 120 ]. However, dietary ingredients and nutritional supplements are diverse and complex in composition, and their modulation of antitumor immune response and contribution to immunotherapy remain to be explored.
The third party of tumor immunity: microbial-related biomarkers
The influence of microorganisms on tumors can be roughly divided into two aspects. For one thing, intestinal microorganisms indirectly affect the progression of tumors mainly through metabolic pathways. For example, gastrointestinal cancer and liver cancer may be regulated by the metabolites of intestinal microorganisms such as bile acids [ 121 ]; In breast cancer, intestinal microorganisms may interfer with steroid metabolism and lead to changes in estrogen spectrum [ 122 ]. Even brain tumors are thought to be influenced by gut microbes via the gut-brain axis [ 123 ]. For another, the microbiota within tumors has also been shown to influence tumor progression and therapeutic response. For example, increased intratumoral microbiota can lead to mutagenesis in tumorigenesis, modulation of carcinogenic pathways, or alterations in the host immune system [ 124 ]. The study has found that intestinal flora can predict the efficacy, change the metabolism of immunotherapy drugs, and affect the bioavailability and efficacy of drugs in the host body [ 125 ]. In general, patients with higher diversity and more stable composition of intestinal flora have better ICT efficacy [ 124 ]. Besides, opportunistic fungi can also influence the immune response through the fungus-sensing protein Dectin-1. Therefore, inhibiting bacteria may impair tumor immunity while inhibiting fungi may enhance immunotherapy efficacy [ 126 ]. However, given the diversity of microbiome and the dynamic changes in microenvironment, as well as the complex links between the microbiome and genetics, environment and diet, the complex interactions between the microbiome and tumor immunity need to be further studied with standardized measures [ 124 ].
Auxiliary regulation of tumor immunity: exercise-related biomarkers
Exercise is an important part of comprehensive treatment of cancer and is related to the amount, time, frequency, and mode of exercise. The study found that moderate-to-vigorous intensity aerobic exercise or late morning aerobic exercise was more effective in improving metabolism and lipolysis, while there was no significant difference in the risk of death from cancer between regular and concentrated daily exercise [ 127 ]. Of note, the effects of exercise in reducing tumor mortality and delaying tumor progression, which is difficult to reverse existing tumors, are achieved primarily by promoting antitumor immunity [ 128 ]. Specifically, exercise enhances the differentiation and recruitment of anti-tumor immune cells by stimulating sympathetic nerves, regulating endocrine levels, accelerating metabolism and even improving mood, which helps relieve immunosuppressive factors such as hypoxia, oxidative stress and high lipid in TME [ 127 ]. For example, aerobic exercise can inhibit the growth of pancreatic cancer in mice by activating the immune system, especially IL-15Rα + CD8 + T cells [ 129 ]. As an indicator of exercise performance, the body’s muscle mass can be used as a potential marker of tumor immunity, and the study has also considered sarcopenia as a marker of poor prognosis of ICT [ 130 ]. The newly generated muscle will secrete many hormones and growth factors during exercise and contraction, which are collectively called myokine [ 131 ]. IL-4/6/7/8/15 helps to maintain the balance between pro-inflammatory and anti-inflammatory mediators and promote the differentiation, recruitment and effect of anti-tumor immune cells. In addition, the increase in actin in the circulation caused by exercise helps to activate the immune system [ 132 ].
Effect of bad living habits on tumor immunity
Unhealthy lifestyle habits, such as smoking, drinking and staying up late, not only have carcinogenic effects, but also have immunosuppressive effects. Smoking or second hand smoke can lead to a variety of cancers [ 133 ]. Interestingly, smokers have a better effect on ICT than non-smokers. The further study has found that cigarette smoke induces the overexpression of PD-L1 through aryl hydrocarbon receptor, which leads to tumor immune escape and sensitivity to ICT [ 134 ]. However, some studies have found that smokers’ tumors highly express tissue resident memory T cells (TRM), which mediates immune escape and is insensitive to immunotherapy by exerting immune pressure on tumors [ 135 ]. Smoking also raises risk for colorectal cancer (CRC), especially in people with low T-cell responses. Similarly, alcohol use, even in moderation, reduces antitumor immune surveillance by mediating DNA damage (acetaldehyde), affecting the metabolism of micronutrients (e.g., folate, vitamins B12, B6, and A), or generating large amounts of free radicals [ 136 ]. In addition, alcohol was found to promote PD-L1 expression on CRC cells through the induction of aldehyde dehydrogenase 2 [ 137 ]. Similarly, it has been demonstrated that the disruption of circadian clock caused by regular stay up will greatly destroy the proportion of immune cells and induce immunosuppressive TME, which will significantly reduce the ability of immune cells to destroy cancer cells and promote the rapid growth of tumors [ 138 ].
Clinical application of tumor immune biomarkers
Tumor immune biomarkers can be roughly divided into two categories. One is tumor-related biomarkers, mainly tumor antigens, which are used to directly target tumor cells. The other is immune-related biomarkers, mainly immune checkpoints (ICs), which are used to modulate the state of the tumor immune response. Tumor specificity largely determines the clinical application value of tumor immune biomarkers [ 139 ].
It is imperative to explore the optimal utilization of biomarkers to enhance their clinical applicability. This encompasses the identification and assessment techniques of markers, the design of drug structures, and the methods of drug delivery. Markers from invasive examination such as biopsy specimens can only reflect the static state of part tumor tissues at a certain point in time due to the heterogeneity of tumor tissues, so the results may be biased or not time-effective [ 140 ]. Although the markers from non-invasive examinations such as blood are convenient to dynamically reflect the overall situation of the tumor in real time, the results are difficult to detect or not accurate enough due to low expression level or many other interfering factors [ 141 ]. To better detect the expression of PD-L1, new liquid detection methods are being studied, including soluble PD-L1 (sPD-L1) [ 142 ], circulating tumor PD-L1 (PD-L1*CTCs) and exosomal PD-L1 [ 143 ]. Other liquid detection methods for tumors based on DNA differences in mature red blood cells are also under the research [ 144 ]. Different from the traditional “pathological biopsy + immunohistochemistry,” which is subject to the dynamic changes and heterogeneity of the target, immuno-positron emission tomography (immuno-PET) is a novel diagnostic technology that is highly specific, sensitive, non-invasive, and provides real-time dynamic imaging in vivo [ 145 ]. By labeling monoclonal antibodies with positron nuclides, immuno-PET combines the targeting specificity of antibodies with the high sensitivity of PET. This approach provides information on tumor immune responses across focal areas, treatment stages, and tumor progression directly by monitoring immune biomarkers such as CTLA-4, PD-1/L1, HER2, and MHC molecules [ 146 , 147 ].
The drug structure about tumor antigen is mostly similar to antibody drug conjugate (ADC), that is, warhead (such as antibody, TCR or chimeric antigen receptor (CAR) targeting tumor antigen) plus ammunition (such as toxin, drug or immune cells killing tumor cells) and the connection structure of the two. The influencing factors include lysability, solubility, drug antibody coupling ratio (DAR) and bystander effect [ 148 ]. The drug structure around ICs is dominated by antibodies, which can be divided into active and blocking types. To increase tumor permeability and reduce immunogenicity, only the Fab segment can be retained, but the Fc segment can mediate the ADCC effect [ 149 ].
Regarding drug administration, considering the attributes of chronic tumor diseases and the requirement for prolonged drug usage, the future trajectory for antitumor drugs lies in subcutaneous intratumoral injection or even oral administration (nano-strategies) [ 150 ]. Furthermore, the integration of bionic nanoparticles with cell membrane coatings (such as erythrocyte membranes and engineered tumor membranes) enhances the tumor-targeting capability and biocompatibility of drugs, building upon the inherent selective permeability and vascular retention properties of conventional nanomaterials [ 151 ]. Similarly, engineered bacteria combined with quorum sensing effects can also help achieve targeted tumor killing [ 152 ].
It is important to acknowledge that the combination of different biomarkers can exert better anti-tumor effect. For instance, the combination of ICD inducers and ICT can stimulate immune infiltration while preserving their anti-tumor capabilities [ 153 ]. ICIs combined with immune adjuvant, such as IL-15 superagonist, can synergistically enhance anti-tumor immune activation [ 154 ]. Additionally, the integration of ICIs with TGF-β antibodies or ECM inhibitors facilitates drug penetration into the tumor [ 155 ]. Biomarkers that target both immune and tumor cells can aid immune cells in approaching tumor cells and initiating the cytotoxic effect. The redirection of engineered immune cells by bispecific antibodies (BsAbs) can reverse the off-target and drug resistance of cellular immunotherapy [ 156 ].
Treatment strategies based on tumor antigens
In comparison to TAA, TSA (especially the epitopes) has higher immunogenicity, closer relationship with tumor and more stable expression, so it is not easy to induce immune tolerance, autoimmune response and drug resistance [ 157 ]. Therefore, TSA has become a highly potential biomarker in tumor antigen-based therapeutic strategies. Due to the strong heterogeneity of TSA, it is difficult to determine the inevitable relationship between a certain mutation type and TSA, and the number of TSA has been identified is far less and more difficult to apply than TAA [ 158 ]. Therefore, we still need to actively search for more TSA shared in tumor patients, such as TSA generated by KRAS G12D2 mutation [ 159 ]. An evaluation of the priority of tumor antigens at different expression sites shows that, based on clinical effect, immunogenicity, specificity, tumor correlation, expression level and positive rate, and cell expression distribution, internal tumor antigens (mainly TSA) are more clinically useful than tumor surface antigens and soluble secreted antigens (mainly TAA) [ 160 ]. However, it is difficult for most antigen-based treatment strategies to directly target antigens inside tumor cells [ 158 ]. Thus, inducing the death of tumor cells contributes to the release of antigens within the tumor and contact with immune cells (Fig. 4 ).
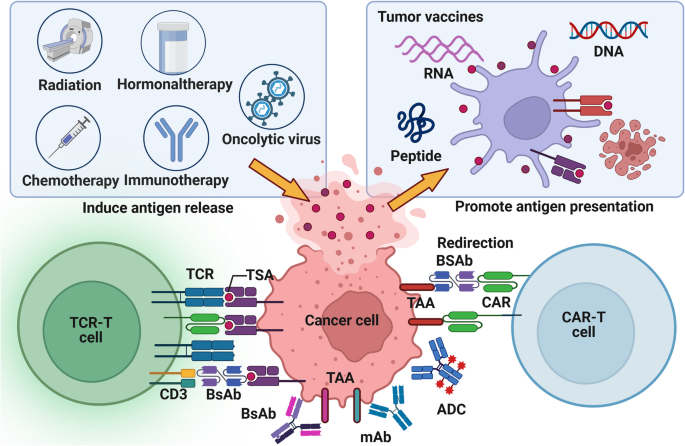
Tumor antigen-based treatment strategies. Tumor antigen-based therapies encompass tumor vaccines, ACT, tumor antibodies, and inducers of ICD. Tumor vaccines emulate the mechanism through which tumor cells release or express tumor antigens, thereby initiating and augmenting anti-tumor T cell immune responses. These vaccines offer many advantages, including eliciting a broad spectrum of anti-tumor immunity, exhibiting high variability, and demonstrating robust efficacy in overcoming tumor heterogeneity. Compared to established vaccines for infectious diseases, tumor vaccines are relatively straightforward and cost-effective to prepare, except for DC vaccines. However, their utilization in clinical settings remains infrequent. Adoptive cell therapy replicates the procedure of screening and amplifying anti-tumor immune cells, resulting in a substantial enhancement in both quantity and efficacy of these cells. The advantages of ACT in anti-tumor immunity encompass robust and enduring response, prompt initiation, heightened specificity, and potent capacity to overcome immunosuppression. Thereby, ACT circumvents the issue of adverse effects associated with excessive immune stimulation. Nevertheless, certain challenges persist, including limited scope of anti-tumor immune response, diminished variability, inadequate ability to surmount tumor heterogeneity, arduous preparation, protracted process and high cost. Synthetic tumor-targeting antibodies emulate the functional mechanism of naturally occurring antibodies in vivo, thus labeling tumor cells, promoting ADCC effect, blocking tumor-promoting receptor signaling, connecting target cells with killer cells, and carrying anti-tumor substances. Tumor antibodies have the advantages of strong anti-tumor immune responses, high specificity, low side effects and relatively simple preparation, especially for hematological tumors. They account for a large part of the researches and development of anticancer drugs and some of them have entered clinical application. ICD inducers promote tumor production of DAMPs to activate anti-tumor innate and adaptive immune responses. The current clinical application of ICD inducers tends to combine them with immunotherapy or targeted drugs for anti-tumor therapy, and to develop new drug delivery methods
Targeting tumor antigens: tumor surface antigens and MHC-bound internal antigens
The therapeutic strategies targeting tumor antigens, especially TSA, mainly include tumor vaccines, adoptive cell therapy (ACT), and tumor antibodies [ 158 ]. For relatively easy-produced and inexpensive tumor vaccines, although the development of preventive vaccines has made significant achievements, most therapeutic vaccines are limited to phase III clinical studies due to low immunogenicity [ 161 ]. Therefore, DC vaccines with high immunogenicity and active antigen presentation may be an important direction for future development, but it is still difficult and expensive to produce [ 162 ]. Traditional tumor vaccines mainly focus on improving the cellular activity of CD8 + T cells, while the screening of tumor antigen dominant CTL epitope peptides is helpful to find new immune active targets that synergistically activate CD8 + T cells, NK cells and DC [ 163 ].
Different from the directly expressed tumor surface antigens, MHC can bind to the intracellular concentrated and processed tumor internal antigens, and express them on the cell surface [ 164 ]. Unlike CAR-T cell therapy and tumor antibodies restricted to tumor surface antigens, TCR-T cell therapy utilizes the immune surveillance mechanism to more sensitively recognize a wider range of tumor internal antigens with low levels of variation and thus play a better anti-tumor effect [ 26 ]. However, the adaptive regulation of MHC expression level and the individualized restriction of MHC make it difficult for TCR-T to be developed as universal as CAR-T/NK cell therapy, and it is not easy to recognize lipid or carbohydrate tumor-related substances [ 165 ]. In addition, both ACT and tumor antibodies are susceptible to the interference of soluble targets and are mistakenly activated in advance (Table 1 ) [ 166 ].
Induction of tumor cell death: release of internal antigens
Unlike the surface antigens of tumors, which are susceptible to the selective pressure of immunotherapy leading to drug resistance, the expression of internal antigens of tumors is more stable and closely associated with the tumor itself [ 167 ]. Although stress challenges of TME can spontaneously lead to the death of tumor cells, the artificially induced ICD of tumor cells is more conducive to the release of internal tumor antigens and the activation of immune infiltration [ 168 ]. ICD inducers have a dual effect of chemotherapy-immunotherapy. However, he effect of ICD induction in tumor cells by a single ICD inducer is usually weak and limited, which is usually achieved by triggering the secondary or “incidental” effect of anticancer drugs, and even instead promotes the formation of immunosuppressive TME [ 169 ]. On the other hand, tumors also evade ICD through various strategies, including the reduction and degradation of ATP release, the reduction of annexin A1 (ANXA1) expression, and the reduction of CRT [ 170 ]. Thus, specific interventions that are based on these defective links, such as induction of autophagy, or in combination with other immunotherapies, such as ICIs and ACT, may restore or enhance the efficacy of ICD inducers.
It is necessary to study the effect of tumor mortality and the immune effect of different ways of death [ 171 ]. According to morphological, biochemical, immunological, and genetic characteristics, regulatory cell death (RCD) can be divided into apoptotic and non-apoptotic types. Apoptosis is considered immune-tolerated, while non-apoptotic RCD can be classified as autophagy, ferroptosis, pyroptosis, and necroptosis. In some cases, non-apoptotic RCD is considered a manifestation of ICD. On one hand, autophagy can inhibit the immune escape of tumors by degrading immune checkpoints, such as the endocytic recycling of PD-L1 [ 172 ]. On the other hand, it can prevent the initiation and activation of anti-tumor T cells by mediating the degradation of MHC class I/II molecules [ 173 ]. Ferroptosis has found to enhance the anti-tumor effect of CD8 + T cells [ 174 ]. Pyroptosis and necroptosis have been shown to promote DAMP release, trigger antigen presentation, and thus activate adaptive immune responses [ 175 ]. Therefore, targeted therapies against autophagy, ferroptosis, pyroptosis, and necroptosis help to convert ICI-resistant “cold” tumors into immunologically active “hot” tumors. However, therapies targeting non-apoptotic RCD may have unintended detrimental effects on tumor-associated immune cells and lead to undesirable toxicity (Table 2 ) [ 176 ].
Treatment strategy based on immune checkpoint
As a crucial regulator of the immune system in healthy individuals, IC plays a pivotal role in preserving autoimmune tolerance and modulating the extent and duration of immune responses. But in the context of tumorigenesis, IC can be exploited by malignant cells, leading to aberrant expression and subsequently facilitating tumor-induced immunosuppression [ 177 ]. Therefore, the general policy of IC-based treatment strategy is to induce new or restore the original anti-tumor immune response, but it usually has problems such as low patient response rate, drug resistance and side effects. In addition to taking key IC as the main therapeutic target, other tumor immune biomarkers are also needed to predict and supervise the response of ICT. These include the gene level (such as microsatellite instability (MSI) and TMB) [ 178 ], the molecular protein level (such as IL-6, chemokines, inflammation and metabolism-related molecules [ 179 ]), the cellular level (such as immune cell frequency, TLS and TDLN), and the microbial level (such as viruses and gut microbes) [ 180 ]. In some cases, the efficacy of ICT and its side effects are complementary, that is, good efficacy is often accompanied by side effects, which in turn can be used as a sign of response to treatment [ 181 ]. Thus, how to balance ICT and its side effects deserves our further study(Fig. 5 ).
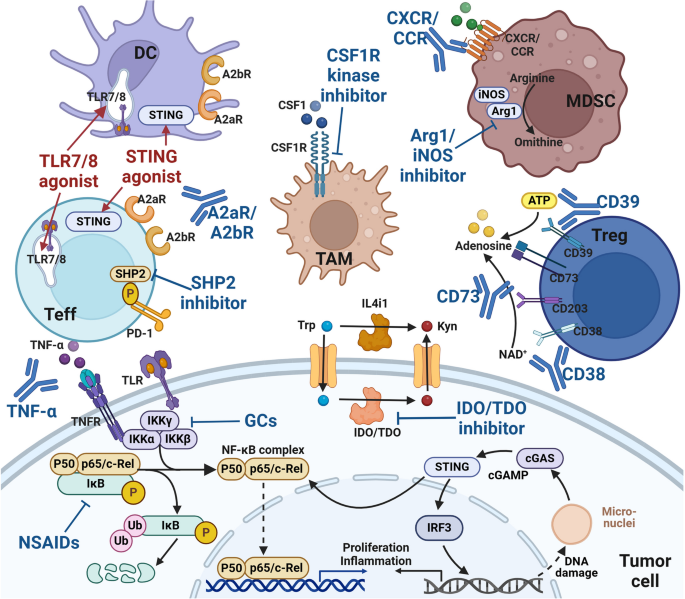
Immune checkpoint-based treatment strategies. Tumor cells actively interact with the surrounding TME and develop various adaptive strategies to form a continuously progressive and highly heterogeneous whole. Various ICs regulate tumor immune responses through a balance of inhibitory and activating signals. In addition to classical cell-surface ICs (such as PD-1/PD-L1 and CTLA-4), they also include extracellular ICs (such as TNF-α and TGF-β), intracellular ICs (such as NF-κB, STING, NR2F6 and LMTK3) and metabolism-related ICs (such as IDO and CD73). More and more ICs pathways have been found to play an important role in driving tumor immune escape. Therefore, ICS pathway may become a promising target for the development of new anticancer immunotherapy
Targeting tumor-local immunity: the activation state of immune cells
A series of ICs that regulate the activation state of immune cells mainly include AKRs and IKRs of NK cells, ‘eat me’ and ‘don’t eat me’ receptors of macrophages, and positive and negative co-stimulatory molecules of T cells. In addition to classic ICs such as PD-1/L1 and CTLA-4, innate immune signals (such as TLR and STING) [ 182 ], adenosine axis signals (such as CD39 and CD73) [ 43 ], metabolic pathways of key amino acids (such as IDO) and downstream signals of TCR (such as tyrosine protein phosphatase non-receptor types (PTPN6 and PTPN22)) are all involved in the regulation of immune cell activation status [ 183 ]. It can be used as the target of novel small molecule drugs. The difference of efficacy and applicable cancer types among different ICs and the interaction between them deserve our attention.
The severity of IRAEs is contingent upon the extent of cellular involvement in ICT expression, the stage of cellular differentiation, and the underlying mechanism of action. For instance, it is noteworthy that red blood cells possess the ability to express the macrophage ‘don’t eat me’ receptor CD47, and the employment of immunotherapy targeting it may result in the development of anemia [ 184 ]. Additionally, the impact of anti-CTLA-4 primarily affects Th0 cells, potentially leading to excessive immune activation. But anti-PD-1 primarily influences Teff cells, thereby facilitating the restoration of immune-mediated anti-tumor effects [ 185 ]. Certain ICIs exhibit a more intricate mechanism of action, potentially exerting a dual effect on tumor cells. Consequently, solely blocking or enhancing their signals may not yield the desired anti-tumor outcome. For instance, as the tumor progresses, tumor cells themselves may develop tolerance to the inhibition of TGF-β, while immune cells remain suppressed due to the abundant secretion of TGF-β. This immune escape of tumors implies that restoring the sensitivity of tumor cells to TGF-β could prove more beneficial in addressing the challenges encountered in TGF-β antibody therapy [ 186 ]. In addition, relevant studies on the endocytosis, recycling, and degradation mechanism of PD-L1 help to make up for the deficiency of PD-L1 antibody therapy (Table 3 ) [ 187 ].
Targeted tumor system immunity: development, differentiation and recruitment of immune cells
Tumor as a systemic disease can remodel function and composition of peripheral immune cells. It is mainly manifested by changes in the frequency of immune cells and their related factors in blood circulation and immune organs, resulting in different responses to immunotherapy in patients with different immune backgrounds [ 72 ]. Tumors have the capacity to remodel the microenvironment of immune organs, primarily bone marrow and TDLN, thereby impeding the maturation of immune cells and promoting the development of immunosuppressive phenotypes [ 188 ]. Additionally, tumors can modulate the expression of chemokines in the bloodstream, leading to the suppression of anti-tumor immune cells while facilitating the migration of pro-tumor immune cells from immune organs to the TME [ 189 ]. In addition to immunotherapy, traditional tumor treatments such as surgery, radiotherapy and chemotherapy can also change the immune background environment of the tumor and affect the therapeutic effect [ 72 ]. Therefore, the general principle of therapeutic strategies targeting tumor systemic immunity is to promote the development, differentiation and recruitment of anti-tumor immune cells or inhibit them of pro-tumor immune cells. However, the specificity and accuracy of artificial regulation of tumor systemic immunity are low (except for targeting colony-stimulating factor 1 (CSF-1) [ 190 ]). At present, it is mainly used to monitor the progress of tumor immunity and predict the effect of immunotherapy by detecting tumor systemic immunity [ 191 ].
Conclusions
In the current review, we comprehensively summarized the war between the immune system and the tumors. As a large battle, ICs provide the necessary trace for the immune system and drugs to accurately attack the tumor. Interestingly, emerging factors significantly affect tumor immunity and thus mediate immunotherapy responses. As a form of physiological intervention, exercise reduces cancer risk and cancer-related morbidity, also re-shape TME via several machineries, should deserve more attentions in future research. Given the complex and diverse regulatory mechanisms of anti-tumor immunity, it is certain that an improved understanding of the unbalance between the immune system and the tumors will provide useful insights into tumorigenicity and may lead to novel clinical applications.
Availability of data and materials
No datasets were generated or analysed during the current study.
Abbreviations
Adoptive cell therapy
Antibody drug conjugate
Antibody-dependent cell-mediated cytotoxic effect
Antibody-dependent cell-mediated phagocytos
Activation induced cell death
Activated killer cell receptor
Antigen presenting cell
Adenosine triphosphate
Regulatory B cells
Bispecific antibodies
Cancer-associated fibroblasts
Cchimeric antigen receptor
Ccomplement-dependent cytotoxicity
Type 1 conventional dendritic cells
Type 2 conventional dendritic cells
Colorectal cancer
Calreticulin
Cold shock domain-containing protein E1
Colony-stimulating factor 1
Cytotoxic T lymphocyte
Cytotoxic T lymphocyte antigen 4
Damage-associated molecular patterns
Drug antibody coupling ratio
Dendritic cells
Extracellular matrix
Erythroid derived myeloid cells
Erythroid progenitor cells
Endoplasmic reticulum
Fatty acid oxidation
Human epidermal growth factor receptor 2
Human endogenous retroviruses
Human leukocyte antigen
High mobility group protein 1
Heat shock protein
Immune checkpoint inhibitors
Immune checkpoints
Immune checkpoint therapy
Indoleamine 2, 3-dioxygenase
Interferon-γ
Inhibitory killer cell receptors
Interleukin-10
Inducible nitric oxide synthase
Immune-related adverse events
interferon regulatory factor 1
Kruppel-like factor 2
Long non-coding RNA
Monoclonal antibodies
Mitogen-activated protein kinase
Marrow-derived suppressor cells
Major histocompatibility complex
Monocyte MDSCs
Mature regulatory dendritic cell
Microsatellite instability
Natural killer cells
Nuclear receptor subfamily Group 2 F-member 6
Programmed death receptor-1
Prostaglandin E2
Peptide-MHC
Polymorphonuclear cells MDSCs
Pattern recognition receptors
Regulatory cell death
Senescence-associated secretory phenotype
Soluble PD-L1
Tumor-associated antigens
Tumor-associated macrophages
T cell receptors
Tumor draining lymph node
Effector T cells
T cell exhaustion
Transforming growth factor β
Initial T cells
Toll-like receptors
Tertiary lymphoid structure
Tumor mutation burden
Tumor microenvironment
Tumor necrosis factor
TNF-related apoptosis-inducing ligand
Regulatory T cells
Tissue resident memory T cells
Tumor-specific antigens
Vascular endothelial growth factor
V-domain Ig suppressor of T cell activation
Vasculogenic mimicry
β2 microglobulin
Helmy KY, Patel SA, Nahas GR, Rameshwar P. Cancer immunotherapy: accomplishments to date and future promise. Ther Deliv. 2013;4(10):1307–20.
Article CAS PubMed Google Scholar
Ribatti D. The concept of immune surveillance against tumors. The first theories. Oncotarget. 2017;8(4):7175–80.
Article PubMed Google Scholar
O’Donnell JS, Teng MWL, Smyth MJ. Cancer immunoediting and resistance to T cell-based immunotherapy. Nat Rev Clin Oncol. 2019;16(3):151–67.
Yi M, Jiao D, Qin S, Chu Q, Wu K, Li A. Synergistic effect of immune checkpoint blockade and anti-angiogenesis in cancer treatment. Mol Cancer. 2019;18(1):60.
Article PubMed PubMed Central Google Scholar
Chu T, Zhong R, Zhong H, Zhang B, Zhang W, Shi C, Qian J, Zhang Y, Chang Q, Zhang X, et al. Phase 1b study of sintilimab plus anlotinib as first-line therapy in patients with advanced NSCLC. J Thorac Oncol. 2021;16(4):643–52.
Fu W, Lei C, Wang C, Ma Z, Li T, Lin F, Mao R, Zhao J, Hu S. Synthetic libraries of immune cells displaying a diverse repertoire of chimaeric antigen receptors as a potent cancer immunotherapy. Nat Biomed Eng. 2022;6(7):842–54.
Ramos-Casals M, Brahmer JR, Callahan MK, Flores-Chávez A, Keegan N, Khamashta MA, Lambotte O, Mariette X, Prat A, Suárez-Almazor ME. Immune-related adverse events of checkpoint inhibitors. Nat Rev Dis Primers. 2020;6(1):38.
Jelski W, Mroczko B. Molecular and circulating biomarkers of gastric cancer. Int J Mol Sci. 2022;23(14):7588.
Article CAS PubMed PubMed Central Google Scholar
Tsimberidou AM, Fountzilas E, Nikanjam M, Kurzrock R. Review of precision cancer medicine: evolution of the treatment paradigm. Cancer Treat Rev. 2020;86:102019.
Pudkasam S, Tangalakis K, Chinlumprasert N, Apostolopoulos V, Stojanovska L. Breast cancer and exercise: the role of adiposity and immune markers. Maturitas. 2017;105:16–22.
Chen DS, Mellman I. Elements of cancer immunity and the cancer-immune set point. Nature. 2017;541(7637):321–30.
Kalaora S, Nagler A, Nejman D, Alon M, Barbolin C, Barnea E, Ketelaars SLC, Cheng K, Vervier K, Shental N, et al. Identification of bacteria-derived HLA-bound peptides in melanoma. Nature. 2021;592(7852):138–43.
Lim RJ, Liu B, Krysan K, Dubinett SM. Lung cancer and immunity markers. Cancer Epidemiol Biomarkers Prev. 2020;29(12):2423–30.
Mashouri L, Yousefi H, Aref AR, Ahadi AM, Molaei F, Alahari SK. Exosomes: composition, biogenesis, and mechanisms in cancer metastasis and drug resistance. Mol Cancer. 2019;18(1):75.
Liu YT, Sun ZJ. Turning cold tumors into hot tumors by improving T-cell infiltration. Theranostics. 2021;11(11):5365–86.
Bradley RK, Anczuków O. RNA splicing dysregulation and the hallmarks of cancer. Nat Rev Cancer. 2023;23(3):135–55.
Li R, Di L, Li J, Fan W, Liu Y, Guo W, Liu W, Liu L, Li Q, Chen L, et al. A body map of somatic mutagenesis in morphologically normal human tissues. Nature. 2021;597(7876):398–403.
Liu J, Peng Y, Wei W. Cell cycle on the crossroad of tumorigenesis and cancer therapy. Trends Cell Biol. 2022;32(1):30–44.
Zhang Z, Lu M, Qin Y, Gao W, Tao L, Su W, Zhong J. Neoantigen: a new breakthrough in tumor immunotherapy. Front Immunol. 2021;12:672356.
Wang J, Xiu J, Farrell A, Baca Y, Arai H, Battaglin F, Kawanishi N, Soni S, Zhang W, Millstein J, et al. Mutational analysis of microsatellite-stable gastrointestinal cancer with high tumour mutational burden: a retrospective cohort study. Lancet Oncol. 2023;24(2):151–61.
Kumar S, Warrell J, Li S, McGillivray PD, Meyerson W, Salichos L, Harmanci A, Martinez-Fundichely A, Chan CWY, Nielsen MM, et al. Passenger mutations in more than 2,500 Cancer genomes: overall molecular functional impact and consequences. Cell. 2020;180(5):915-927.e916.
Sherman MA, Yaari AU, Priebe O, Dietlein F, Loh PR, Berger B. Genome-wide mapping of somatic mutation rates uncovers drivers of cancer. Nat Biotechnol. 2022;40(11):1634–43.
Burbage M, Rocañín-Arjó A, Baudon B, Arribas YA, Merlotti A, Rookhuizen DC, Heurtebise-Chrétien S, Ye M, Houy A, Burgdorf N, et al. Epigenetically controlled tumor antigens derived from splice junctions between exons and transposable elements. Sci Immunol. 2023;8(80):eabm6360.
Chen F, Zou Z, Du J, Su S, Shao J, Meng F, Yang J, Xu Q, Ding N, Yang Y, et al. Neoantigen identification strategies enable personalized immunotherapy in refractory solid tumors. J Clin Invest. 2019;129(5):2056–70.
Jiang T, Shi T, Zhang H, Hu J, Song Y, Wei J, Ren S, Zhou C. Tumor neoantigens: from basic research to clinical applications. J Hematol Oncol. 2019;12(1):93.
He J, Xiong X, Yang H, Li D, Liu X, Li S, Liao S, Chen S, Wen X, Yu K, et al. Defined tumor antigen-specific T cells potentiate personalized TCR-T cell therapy and prediction of immunotherapy response. Cell Res. 2022;32(6):530–42.
Majumder A, Sandhu M, Banerji D, Steri V, Olshen A, Moasser MM. The role of HER2 and HER3 in HER2-amplified cancers beyond breast cancers. Sci Rep. 2021;11(1):9091.
Lowery FJ, Krishna S, Yossef R, Parikh NB, Chatani PD, Zacharakis N, Parkhurst MR, Levin N, Sindiri S, Sachs A, et al. Molecular signatures of antitumor neoantigen-reactive T cells from metastatic human cancers. Science. 2022;375(6583):877–84.
Naghavian R, Faigle W, Oldrati P, Wang J, Toussaint NC, Qiu Y, Medici G, Wacker M, Freudenmann LK, Bonté PE, et al. Microbial peptides activate tumour-infiltrating lymphocytes in glioblastoma. Nature. 2023;617(7962):807–17.
Canale FP, Basso C, Antonini G, Perotti M, Li N, Sokolovska A, Neumann J, James MJ, Geiger S, Jin W, et al. Metabolic modulation of tumours with engineered bacteria for immunotherapy. Nature. 2021;598(7882):662–6.
Jakobsson J, Vincendeau M. SnapShot: human endogenous retroviruses. Cell. 2022;185(2):400-e400401.
Koch U, Radtke F. Mechanisms of T cell development and transformation. Annu Rev Cell Dev Biol. 2011;27:539–62.
Kroemer G, Galassi C, Zitvogel L, Galluzzi L. Immunogenic cell stress and death. Nat Immunol. 2022;23(4):487–500.
Krysko DV, Garg AD, Kaczmarek A, Krysko O, Agostinis P, Vandenabeele P. Immunogenic cell death and DAMPs in cancer therapy. Nat Rev Cancer. 2012;12(12):860–75.
Marin I, Boix O, Garcia-Garijo A, Sirois I, Caballe A, Zarzuela E, Ruano I, Attolini CS, Prats N, López-Domínguez JA, et al. Cellular senescence is immunogenic and promotes antitumor Immunity. Cancer Discov. 2023;13(2):410–31.
Kroemer G, Galluzzi L, Kepp O, Zitvogel L. Immunogenic cell death in cancer therapy. Annu Rev Immunol. 2013;31:51–72.
Lv J, Zhou Y, Zhou N, Wang Z, Chen J, Chen H, Wang D, Zhou L, Wei K, Zhang H, et al. Epigenetic modification of CSDE1 locus dictates immune recognition of nascent tumorigenic cells. Sci Transl Med. 2023;15(681):eabq6024.
Peng F, Liao M, Qin R, Zhu S, Peng C, Fu L, Chen Y, Han B. Regulated cell death (RCD) in cancer: key pathways and targeted therapies. Signal Transduct Target Ther. 2022;7(1):286.
Huang J, Xie Y, Sun X, Zeh HJ 3, Kang R, Lotze MT, Tang D. DAMPs, ageing, and cancer: the ‘DAMP hypothesis.’ Ageing Res Rev. 2015;24(Pt A):3–16.
Eil R, Vodnala SK, Clever D, Klebanoff CA, Sukumar M, Pan JH, Palmer DC, Gros A, Yamamoto TN, Patel SJ, et al. Ionic immune suppression within the tumour microenvironment limits T cell effector function. Nature. 2016;537(7621):539–43.
Cilenti F, Barbiera G, Caronni N, Iodice D, Montaldo E, Barresi S, Lusito E, Cuzzola V, Vittoria FM, Mezzanzanica L, et al. A PGE(2)-MEF2A axis enables context-dependent control of inflammatory gene expression. Immunity. 2021;54(8):1665-1682.e1614.
Chen X, Kang R, Kroemer G, Tang D. Broadening horizons: the role of ferroptosis in cancer. Nat Rev Clin Oncol. 2021;18(5):280–96.
Yegutkin GG, Boison D. ATP and adenosine metabolism in cancer: exploitation for therapeutic gain. Pharmacol Rev. 2022;74(3):797–822.
Man SM, Jenkins BJ. Context-dependent functions of pattern recognition receptors in cancer. Nat Rev Cancer. 2022;22(7):397–413.
Maiorino L, Daßler-Plenker J, Sun L, Egeblad M. Innate immunity and cancer pathophysiology. Annu Rev Pathol. 2022;17:425–57.
Li K, Shi H, Zhang B, Ou X, Ma Q, Chen Y, Shu P, Li D, Wang Y. Myeloid-derived suppressor cells as immunosuppressive regulators and therapeutic targets in cancer. Signal Transduct Target Ther. 2021;6(1):362.
Long H, Jia Q, Wang L, Fang W, Wang Z, Jiang T, Zhou F, Jin Z, Huang J, Zhou L, et al. Tumor-induced erythroid precursor-differentiated myeloid cells mediate immunosuppression and curtail anti-PD-1/PD-L1 treatment efficacy. Cancer Cell. 2022;40(6):674-e693677.
Gunesch JT, Dixon AL, Ebrahim TA, Berrien-Elliott MM, Tatineni S, Kumar T, Hegewisch-Solloa E, Fehniger TA, Mace EM. CD56 regulates human NK cell cytotoxicity through Pyk2. Elife. 2020;9:9.
Article Google Scholar
Merino AM, Kim H, Miller JS, Cichocki F. Unraveling exhaustion in adaptive and conventional NK cells. J Leukoc Biol. 2020;108(4):1361–8.
Bald T, Krummel MF, Smyth MJ, Barry KC. The NK cell-cancer cycle: advances and new challenges in NK cell-based immunotherapies. Nat Immunol. 2020;21(8):835–47.
Tarannum M, Romee R, Shapiro RM. Innovative strategies to improve the clinical application of NK Cell-based immunotherapy. Front Immunol. 2022;13:859177.
Myers JA, Miller JS. Exploring the NK cell platform for cancer immunotherapy. Nat Rev Clin Oncol. 2021;18(2):85–100.
Bossard C, Bézieau S, Matysiak-Budnik T, Volteau C, Laboisse CL, Jotereau F, Mosnier JF. HLA-E/β2 microglobulin overexpression in colorectal cancer is associated with recruitment of inhibitory immune cells and tumor progression. Int J Cancer. 2012;131(4):855–63.
Pereira BI, Devine OP, Vukmanovic-Stejic M, Chambers ES, Subramanian P, Patel N, Virasami A, Sebire NJ, Kinsler V, Valdovinos A, et al. Senescent cells evade immune clearance via HLA-E-mediated NK and CD8(+) T cell inhibition. Nat Commun. 2019;10(1):2387.
An Y, Yang Q. Tumor-associated macrophage-targeted therapeutics in ovarian cancer. Int J Cancer. 2021;149(1):21–30.
Wang H, Tian T, Zhang J. Tumor-associated macrophages (TAMs) in colorectal cancer (CRC): from mechanism to therapy and prognosis. Int J Mol Sci. 2021;22(16):8470.
Locati M, Curtale G, Mantovani A. Diversity, mechanisms, and significance of macrophage plasticity. Annu Rev Pathol. 2020;15:123–47.
DeNardo DG, Ruffell B. Macrophages as regulators of tumour immunity and immunotherapy. Nat Rev Immunol. 2019;19(6):369–82.
Wculek SK, Cueto FJ, Mujal AM, Melero I, Krummel MF, Sancho D. Dendritic cells in cancer immunology and immunotherapy. Nat Rev Immunol. 2020;20(1):7–24.
Jhunjhunwala S, Hammer C, Delamarre L. Antigen presentation in cancer: insights into tumour immunogenicity and immune evasion. Nat Rev Cancer. 2021;21(5):298–312.
Green DR, Droin N, Pinkoski M. Activation-induced cell death in T cells. Immunol Rev. 2003;193:70–81.
Ghislat G, Cheema AS, Baudoin E, Verthuy C, Ballester PJ, Crozat K, Attaf N, Dong C, Milpied P, Malissen B, et al. NF-κB-dependent IRF1 activation programs cDC1 dendritic cells to drive antitumor immunity. Sci Immunol. 2021;6(61):eabg3570.
du Bois H, Heim TA, Lund AW. Tumor-draining lymph nodes: at the crossroads of metastasis and immunity. Sci Immunol. 2021;6(63):eabg3551.
Combes AJ, Samad B, Tsui J, Chew NW, Yan P, Reeder GC, Kushnoor D, Shen A, Davidson B, Barczak AJ, et al. Discovering dominant tumor immune archetypes in a pan-cancer census. Cell. 2022;185(1):184-203.e119.
Schenkel JM, Herbst RH, Canner D, Li A, Hillman M, Shanahan SL, Gibbons G, Smith OC, Kim JY, Westcott P, et al. Conventional type I dendritic cells maintain a reservoir of proliferative tumor-antigen specific TCF-1(+) CD8(+) T cells in tumor-draining lymph nodes. Immunity. 2021;54(10):2338-2353.e2336.
Im K, Combes AJ, Spitzer MH, Satpathy AT, Krummel MF. Archetypes of checkpoint-responsive immunity. Trends Immunol. 2021;42(11):960–74.
Maier B, Leader AM, Chen ST, Tung N, Chang C, LeBerichel J, Chudnovskiy A, Maskey S, Walker L, Finnigan JP, et al. A conserved dendritic-cell regulatory program limits antitumour immunity. Nature. 2020;580(7802):257–62.
Vesely MD, Kershaw MH, Schreiber RD, Smyth MJ. Natural innate and adaptive immunity to cancer. Annu Rev Immunol. 2011;29:235–71.
Su S, Zhao J, Xing Y, Zhang X, Liu J, Ouyang Q, Chen J, Su F, Liu Q, Song E. Immune checkpoint inhibition overcomes ADCP-Induced Immunosuppression by macrophages. Cell. 2018;175(2):442-457.e423.
Wouters MCA, Nelson BH. Prognostic significance of tumor-infiltrating B cells and plasma cells in human cancer. Clin Cancer Res. 2018;24(24):6125–35.
Laumont CM, Banville AC, Gilardi M, Hollern DP, Nelson BH. Tumour-infiltrating B cells: immunological mechanisms, clinical impact and therapeutic opportunities. Nat Rev Cancer. 2022;22(7):414–30.
Hiam-Galvez KJ, Allen BM, Spitzer MH. Systemic immunity in cancer. Nat Rev Cancer. 2021;21(6):345–59.
Connolly KA, Kuchroo M, Venkat A, Khatun A, Wang J, William I, Hornick NI, Fitzgerald BL, Damo M, Kasmani MY, et al. A reservoir of stem-like CD8(+) T cells in the tumor-draining lymph node preserves the ongoing antitumor immune response. Sci Immunol. 2021;6(64):eabg7836.
Märkl F, Huynh D, Endres S, Kobold S. Utilizing chemokines in cancer immunotherapy. Trends Cancer. 2022;8(8):670–82.
Ozga AJ, Chow MT, Luster AD. Chemokines and the immune response to cancer. Immunity. 2021;54(5):859–74.
Propper DJ, Balkwill FR. Harnessing cytokines and chemokines for cancer therapy. Nat Rev Clin Oncol. 2022;19(4):237–53.
Verginadis II, Avgousti H, Monslow J, Skoufos G, Chinga F, Kim K, Leli NM, Karagounis IV, Bell BI, Velalopoulou A, et al. A stromal integrated stress response activates perivascular cancer-associated fibroblasts to drive angiogenesis and tumour progression. Nat Cell Biol. 2022;24(6):940–53.
Hamzah J, Jugold M, Kiessling F, Rigby P, Manzur M, Marti HH, Rabie T, Kaden S, Gröne HJ, Hämmerling GJ, et al. Vascular normalization in Rgs5-deficient tumours promotes immune destruction. Nature. 2008;453(7193):410–4.
Chen Y, Yang S, Tavormina J, Tampe D, Zeisberg M, Wang H, Mahadevan KK, Wu CJ, Sugimoto H, Chang CC, et al. Oncogenic collagen I homotrimers from cancer cells bind to α3β1 integrin and impact tumor microbiome and immunity to promote pancreatic cancer. Cancer Cell. 2022;40(8):818-834.e819.
Mao X, Xu J, Wang W, Liang C, Hua J, Liu J, Zhang B, Meng Q, Yu X, Shi S. Crosstalk between cancer-associated fibroblasts and immune cells in the tumor microenvironment: new findings and future perspectives. Mol Cancer. 2021;20(1):131.
Munoz-Erazo L, Rhodes JL, Marion VC, Kemp RA. Tertiary lymphoid structures in cancer - considerations for patient prognosis. Cell Mol Immunol. 2020;17(6):570–5.
Schumacher TN, Thommen DS. Tertiary lymphoid structures in cancer. Science. 2022;375(6576):eabf9419.
Huang Q, Wu X, Wang Z, Chen X, Wang L, Lu Y, Xiong D, Liu Q, Tian Y, Lin H, et al. The primordial differentiation of tumor-specific memory CD8(+) T cells as bona fide responders to PD-1/PD-L1 blockade in draining lymph nodes. Cell. 2022;185(22):4049-4066.e4025.
Helmink BA, Reddy SM, Gao J, Zhang S, Basar R, Thakur R, Yizhak K, Sade-Feldman M, Blando J, Han G, et al. B cells and tertiary lymphoid structures promote immunotherapy response. Nature. 2020;577(7791):549–55.
Yang H, Sun B, Ma W, Fan L, Xu K, Jia Y, Xu J, Wang Z, Yao F. Multi-scale characterization of tumor-draining lymph nodes in resectable lung cancer treated with neoadjuvant immune checkpoint inhibitors. EBioMedicine. 2022;84:104265.
Prokhnevska N, Cardenas MA, Valanparambil RM, Sobierajska E, Barwick BG, Jansen C, Reyes Moon A, Gregorova P, delBalzo L, Greenwald R, et al. CD8(+) T cell activation in cancer comprises an initial activation phase in lymph nodes followed by effector differentiation within the tumor. Immunity. 2023;56(1):107-124.e105.
Weigelin B, den Boer AT, Wagena E, Broen K, Dolstra H, de Boer RJ, Figdor CG, Textor J, Friedl P. Cytotoxic T cells are able to efficiently eliminate cancer cells by additive cytotoxicity. Nat Commun. 2021;12(1):5217.
Zeng Z, Gu SS, Ouardaoui N, Tymm C, Yang L, Wong CJ, Li D, Zhang W, Wang X, Weirather JL, et al. Hippo signaling pathway regulates cancer cell-intrinsic MHC-II expression. Cancer Immunol Res. 2022;10(12):1559–69.
Oh DY, Kwek SS, Raju SS, Li T, McCarthy E, Chow E, Aran D, Ilano A, Pai CS, Rancan C, et al. Intratumoral CD4(+) T cells mediate anti-tumor cytotoxicity in human bladder cancer. Cell. 2020;181(7):1612-1625.e1613.
Takeuchi A, Saito T. CD4 CTL, a cytotoxic subset of CD4(+) T cells, their differentiation and function. Front Immunol. 2017;8:194.
Juno JA, van Bockel D, Kent SJ, Kelleher AD, Zaunders JJ, Munier CM. Cytotoxic CD4 T cells-friend or foe during viral infection? Front Immunol. 2017;8:19.
ElTanbouly MA, Zhao Y, Nowak E, Li J, Schaafsma E, Le Mercier I, Ceeraz S, Lines JL, Peng C, Carriere C, et al. VISTA is a checkpoint regulator for naïve T cell quiescence and peripheral tolerance. Science. 2020;367(6475):eaay0524.
Woodham AW, Yan L, Skeate JG, van der Veen D, Brand HH, Wong MK, Da Silva DM, Kast WM. T cell ignorance is bliss: T cells are not tolerized by Langerhans cells presenting human papillomavirus antigens in the absence of costimulation. Papillomavirus Res. 2016;2:21–30.
Crespo J, Sun H, Welling TH, Tian Z, Zou W. T cell anergy, exhaustion, senescence, and stemness in the tumor microenvironment. Curr Opin Immunol. 2013;25(2):214–21.
Garris CS, Arlauckas SP, Kohler RH, Trefny MP, Garren S, Piot C, Engblom C, Pfirschke C, Siwicki M, Gungabeesoon J, et al. Successful anti-PD-1 cancer immunotherapy requires T cell-dendritic cell crosstalk involving the cytokines IFN-γ and IL-12. Immunity. 2018;49(6):1148-1161.e1147.
Zhao Y, Caron C, Chan YY, Lee CK, Xu X, Zhang J, Masubuchi T, Wu C, Bui JD, Hui E. cis-B7:CD28 interactions at invaginated synaptic membranes provide CD28 co-stimulation and promote CD8(+) T cell function and anti-tumor immunity. Immunity. 2023;56(6):1187-1203.e1112.
ElTanbouly MA, Noelle RJ. Rethinking peripheral T cell tolerance: checkpoints across a T cell’s journey. Nat Rev Immunol. 2021;21(4):257–67.
Ford BR, Vignali PDA, Rittenhouse NL, Scharping NE, Peralta R, Lontos K, Frisch AT, Delgoffe GM, Poholek AC. Tumor microenvironmental signals reshape chromatin landscapes to limit the functional potential of exhausted T cells. Sci Immunol. 2022;7(74):eabj9123.
Khan O, Giles JR, McDonald S, Manne S, Ngiow SF, Patel KP, Werner MT, Huang AC, Alexander KA, Wu JE, et al. TOX transcriptionally and epigenetically programs CD8(+) T cell exhaustion. Nature. 2019;571(7764):211–8.
Beltra JC, Manne S, Abdel-Hakeem MS, Kurachi M, Giles JR, Chen Z, Casella V, Ngiow SF, Khan O, Huang YJ, et al. Developmental relationships of four exhausted CD8(+) T cell subsets reveals underlying transcriptional and epigenetic landscape control mechanisms. Immunity. 2020;52(5):825-841.e828.
Tsui C, Kretschmer L, Rapelius S, Gabriel SS, Chisanga D, Knöpper K, Utzschneider DT, Nüssing S, Liao Y, Mason T, et al. MYB orchestrates T cell exhaustion and response to checkpoint inhibition. Nature. 2022;609(7926):354–60.
Dähling S, Mansilla AM, Knöpper K, Grafen A, Utzschneider DT, Ugur M, Whitney PG, Bachem A, Arampatzi P, Imdahl F, et al. Type 1 conventional dendritic cells maintain and guide the differentiation of precursors of exhausted T cells in distinct cellular niches. Immunity. 2022;55(4):656-670.e658.
Xie F, Zhou X, Li H, Su P, Liu S, Li R, Zou J, Wei X, Pan C, Zhang Z, et al. USP8 promotes cancer progression and extracellular vesicle-mediated CD8 + T cell exhaustion by deubiquitinating the TGF-β receptor TβRII. EMBO J. 2022;41(16):e108791.
Cardoso Alves L, Corazza N, Micheau O, Krebs P. The multifaceted role of TRAIL signaling in cancer and immunity. FEBS J. 2021;288(19):5530–54.
Zhou Q, Zhou L, Qian J, Yuan ZL, Chen ZJ. NKILA inhibition protects retinal pigment epithelium cells from hypoxia by facilitating NFκB activation. Biochem Biophys Res Commun. 2018;503(4):3134–41.
Jiang N, Schonnesen AA, Ma KY. Ushering in integrated T cell repertoire profiling in cancer. Trends Cancer. 2019;5(2):85–94.
Xia L, Oyang L, Lin J, Tan S, Han Y, Wu N, Yi P, Tang L, Pan Q, Rao S, et al. The cancer metabolic reprogramming and immune response. Mol Cancer. 2021;20(1):28.
Vaupel P, Schmidberger H, Mayer A. The Warburg effect: essential part of metabolic reprogramming and central contributor to cancer progression. Int J Radiat Biol. 2019;95(7):912–9.
Wang Z, Dong C. Gluconeogenesis in cancer: function and regulation of PEPCK, FBPase, and G6Pase. Trends Cancer. 2019;5(1):30–45.
Yang K, Wang X, Song C, He Z, Wang R, Xu Y, Jiang G, Wan Y, Mei J, Mao W. The role of lipid metabolic reprogramming in tumor microenvironment. Theranostics. 2023;13(6):1774–808.
Anderson PM, Lalla RV. Glutamine for amelioration of radiation and chemotherapy associated mucositis during cancer therapy. Nutrients. 2020;12(6):1675.
Fujiwara Y, Kato S, Nesline MK, Conroy JM, DePietro P, Pabla S, Kurzrock R. Indoleamine 2,3-dioxygenase (IDO) inhibitors and cancer immunotherapy. Cancer Treat Rev. 2022;110:102461.
Mao W, Cai Y, Chen D, Jiang G, Xu Y, Chen R, Wang F, Wang X, Zheng M, Zhao X, et al. Statin shapes inflamed tumor microenvironment and enhances immune checkpoint blockade in non-small cell lung cancer. JCI Insight. 2022;7(18):e161940.
Salt IP, Nunes JRC, Fullerton MD. Metformin again? Atheroprotection mediated by macrophage AMPK and ATF1. Cardiovasc Res. 2021;117(5):1233–4.
Mohammadifard N, Humphries KH, Gotay C, Mena-Sánchez G, Salas-Salvadó J, Esmaillzadeh A, Ignaszewski A, Sarrafzadegan N. Trace minerals intake: risks and benefits for cardiovascular health. Crit Rev Food Sci Nutr. 2019;59(8):1334–46.
Ozturk M, Metin M, Altay V, Bhat RA, Ejaz M, Gul A, Unal BT, Hasanuzzaman M, Nibir L, Nahar K, et al. Arsenic and human health: genotoxicity, epigenomic effects, and cancer signaling. Biol Trace Elem Res. 2022;200(3):988–1001.
Skrajnowska D, Bobrowska-Korczak B. Role of zinc in immune system and anti-cancer defense mechanisms. Nutrients. 2019;11(10):2273.
Zhao L, Zhou X, Xie F, Zhang L, Yan H, Huang J, Zhang C, Zhou F, Chen J, Zhang L. Ferroptosis in cancer and cancer immunotherapy. Cancer Commun (Lond). 2022;42(2):88–116.
Cimmino L, Neel BG, Aifantis I. Vitamin C in stem cell reprogramming and cancer. Trends Cell Biol. 2018;28(9):698–708.
Yang CS, Luo P, Zeng Z, Wang H, Malafa M, Suh N. Vitamin E and cancer prevention: studies with different forms of tocopherols and tocotrienols. Mol Carcinog. 2020;59(4):365–89.
Lee PC, Wu CJ, Hung YW, Lee CJ, Chi CT, Lee IC, Yu-Lun K, Chou SH, Luo JC, Hou MC, et al. Gut microbiota and metabolites associate with outcomes of immune checkpoint inhibitor-treated unresectable hepatocellular carcinoma. J Immunother Cancer. 2022;10(6):e004779.
Nejman D, Livyatan I, Fuks G, Gavert N, Zwang Y, Geller LT, Rotter-Maskowitz A, Weiser R, Mallel G, Gigi E, et al. The human tumor microbiome is composed of tumor type-specific intracellular bacteria. Science. 2020;368(6494):973–80.
Campbell C, Kandalgaonkar MR, Golonka RM, Yeoh BS, Vijay-Kumar M, Saha P. Crosstalk between gut microbiota and host immunity: impact on inflammation and immunotherapy. Biomedicines. 2023;11(2):294.
Galeano Niño JL, Wu H, LaCourse KD, Kempchinsky AG, Baryiames A, Barber B, Futran N, Houlton J, Sather C, Sicinska E, et al. Effect of the intratumoral microbiota on spatial and cellular heterogeneity in cancer. Nature. 2022;611(7937):810–7.
Si H, Yang Q, Hu H, Ding C, Wang H, Lin X. Colorectal cancer occurrence and treatment based on changes in intestinal flora. Semin Cancer Biol. 2021;70:3–10.
Shiao SL, Kershaw KM, Limon JJ, You S, Yoon J, Ko EY, Guarnerio J, Potdar AA, McGovern DPB, Bose S, et al. Commensal bacteria and fungi differentially regulate tumor responses to radiation therapy. Cancer Cell. 2021;39(9):1202-1213.e1206.
Cao C, Friedenreich CM, Yang L. Association of daily sitting time and leisure-time physical activity with survival among US cancer survivors. JAMA Oncol. 2022;8(3):395–403.
Lee DH, Rezende LFM, Joh HK, Keum N, Ferrari G, Rey-Lopez JP, Rimm EB, Tabung FK, Giovannucci EL. Long-term leisure-time physical activity intensity and all-cause and cause-specific mortality: a prospective cohort of US adults. Circulation. 2022;146(7):523–34.
Kurz E, Hirsch CA, Dalton T, Shadaloey SA, Khodadadi-Jamayran A, Miller G, Pareek S, Rajaei H, Mohindroo C, Baydogan S, et al. Exercise-induced engagement of the IL-15/IL-15Rα axis promotes anti-tumor immunity in pancreatic cancer. Cancer Cell. 2022;40(7):720-737.e725.
Richards SJG, Senadeera SC, Frizelle FA. Sarcopenia, as assessed by Psoas cross-sectional area, is predictive of adverse postoperative outcomes in patients undergoing colorectal cancer surgery. Dis Colon Rectum. 2020;63(6):807–15.
Huang Q, Wu M, Wu X, Zhang Y, Xia Y. Muscle-to-tumor crosstalk: the effect of exercise-induced myokine on cancer progression. Biochim Biophys Acta Rev Cancer. 2022;1877(5):188761.
Kim JS, Wilson RL, Taaffe DR, Galvão DA, Gray E, Newton RU. Myokine expression and tumor-suppressive effect of serum after 12 wk of exercise in prostate cancer patients on ADT. Med Sci Sports Exerc. 2022;54(2):197–205.
Idris S, Baqays A, Isaac A, Chau JKM, Calhoun KH, Seikaly H. The effect of second hand smoke in patients with squamous cell carcinoma of the head and neck. J Otolaryngol Head Neck Surg. 2019;48(1):33.
Wang GZ, Zhang L, Zhao XC, Gao SH, Qu LW, Yu H, Fang WF, Zhou YC, Liang F, Zhang C, et al. The Aryl hydrocarbon receptor mediates tobacco-induced PD-L1 expression and is associated with response to immunotherapy. Nat Commun. 2019;10(1):1125.
Weeden CE, Gayevskiy V, Marceaux C, Batey D, Tan T, Yokote K, Ribera NT, Clatch A, Christo S, Teh CE, et al. Early immune pressure initiated by tissue-resident memory T cells sculpts tumor evolution in non-small cell lung cancer. Cancer Cell. 2023;41(5):837-852.e836.
Rumgay H, Murphy N, Ferrari P, Soerjomataram I. Alcohol and cancer: epidemiology and biological mechanisms. Nutrients. 2021;13(9):3173.
Zhou X, Xiao Q, Jiang F, Sun J, Wang L, Yu L, Zhou Y, Zhao J, Zhang H, Yuan S, et al. Dissecting the pathogenic effects of smoking and its hallmarks in blood DNA methylation on colorectal cancer risk. Br J Cancer. 2023;129(8):1306–13.
Aiello I, Fedele MLM, Román F, Marpegan L, Caldart C, Chiesa JJ, Golombek DA, Finkielstein CV, Paladino N. Circadian disruption promotes tumor-immune microenvironment remodeling favoring tumor cell proliferation. Sci Adv. 2020;6(42):eaaz4530.
Pan Y, Fu Y, Zeng Y, Liu X, Peng Y, Hu C, Deng C, Qiu Z, Zou J, Liu Y, et al. The key to immunotherapy: how to choose better therapeutic biomarkers for patients with non-small cell lung cancer. Biomark Res. 2022;10(1):9.
Vaidyanathan R, Soon RH, Zhang P, Jiang K, Lim CT. Cancer diagnosis: from tumor to liquid biopsy and beyond. Lab Chip. 2018;19(1):11–34.
PubMed Google Scholar
Li W, Liu JB, Hou LK, Yu F, Zhang J, Wu W, Tang XM, Sun F, Lu HM, Deng J, et al. Liquid biopsy in lung cancer: significance in diagnostics, prediction, and treatment monitoring. Mol Cancer. 2022;21(1):25.
Cubillos-Zapata C, Martínez-García M, Campos-Rodríguez F, de la Sánchez M, Nagore E, Martorell-Calatayud A, Hernández Blasco L, Chiner Vives E, Abad-Capa J, Montserrat JM, et al. Soluble PD-L1 is a potential biomarker of cutaneous melanoma aggressiveness and metastasis in obstructive sleep apnoea patients. Eur Respir J. 2019;53(2):1801298.
Ilié M, Szafer-Glusman E, Hofman V, Chamorey E, Lalvée S, Selva E, Leroy S, Marquette CH, Kowanetz M, Hedge P, et al. Detection of PD-L1 in circulating tumor cells and white blood cells from patients with advanced non-small-cell lung cancer. Ann Oncol. 2018;29(1):193–9.
Liang N, Jiao Z, Zhang C, Wu Y, Wang T, Li S, Wang Y, Song T, Chen JQ, Liang H, et al. Mature red blood cells contain long DNA fragments and could acquire DNA from lung cancer tissue. Adv Sci (Weinh). 2023;10(7):e2206361.
Menzies AM, Lastoria S. PET imaging for cancer immunotherapy: the immuno-PET. Ann Oncol. 2022;33(1):13–4.
Sharma G, Braga MC, Da Pieve C, Szopa W, Starzetz T, Plate KH, Kaspera W, Kramer-Marek G. Immuno-PET imaging of tumour PD-L1 expression in glioblastoma. Cancers (Basel). 2023;15(12):3131.
Rothlauf PW, Li Z, Pishesha N, Xie YJ, Woodham AW, Bousbaine D, Kolifrath SC, Verschoor VL, Ploegh HL. Noninvasive immuno-PET imaging of CD8(+) T cell behavior in influenza A virus-infected mice. Front Immunol. 2021;12:777739.
Fu Z, Li S, Han S, Shi C, Zhang Y. Antibody drug conjugate: the “biological missile” for targeted cancer therapy. Signal Transduct Target Ther. 2022;7(1):93.
Iizuka A, Nonomura C, Ashizawa T, Kondou R, Ohshima K, Sugino T, Mitsuya K, Hayashi N, Nakasu Y, Maruyama K, et al. A T-cell-engaging B7-H4/CD3-bispecific fab-scFv antibody targets human breast cancer. Clin Cancer Res. 2019;25(9):2925–34.
Quadir SS, Saharan V, Choudhary D, Harish, Jain CP, Joshi G. Nano-strategies as oral drug delivery platforms for treatment of cancer: challenges and future perspectives. AAPS PharmSciTech. 2022;23(5):152.
Yin T, Diao Z, Blum NT, Qiu L, Ma A, Huang P. Engineering bacteria and bionic bacterial derivatives with nanoparticles for cancer therapy. Small. 2022;18(12):e2104643.
Gurbatri CR, Arpaia N, Danino T. Engineering bacteria as interactive cancer therapies. Science. 2022;378(6622):858–64.
Procureur A, Simonaggio A, Bibault JE, Oudard S, Vano YA. Enhance the Immune checkpoint inhibitors efficacy with radiotherapy induced immunogenic cell death: a comprehensive review and latest developments. Cancers (Basel). 2021;13(4):678.
Wrangle JM, Velcheti V, Patel MR, Garrett-Mayer E, Hill EG, Ravenel JG, Miller JS, Farhad M, Anderton K, Lindsey K, et al. ALT-803, an IL-15 superagonist, in combination with nivolumab in patients with metastatic non-small cell lung cancer: a non-randomised, open-label, phase 1b trial. Lancet Oncol. 2018;19(5):694–704.
Yi M, Li T, Niu M, Wu Y, Zhao Z, Wu K. TGF-β: a novel predictor and target for anti-PD-1/PD-L1 therapy. Front Immunol. 2022;13:1061394.
Piccione EC, Juarez S, Liu J, Tseng S, Ryan CE, Narayanan C, Wang L, Weiskopf K, Majeti R. A bispecific antibody targeting CD47 and CD20 selectively binds and eliminates dual antigen expressing lymphoma cells. MAbs. 2015;7(5):946–56.
Peri A, Salomon N, Wolf Y, Kreiter S, Diken M, Samuels Y. The landscape of T cell antigens for cancer immunotherapy. Nat Cancer. 2023;4(7):937–54.
Xie N, Shen G, Gao W, Huang Z, Huang C, Fu L. Neoantigens: promising targets for cancer therapy. Signal Transduct Target Ther. 2023;8(1):9.
Zhang Q, Jia Q, Zhang J, Zhu B. Neoantigens in precision cancer immunotherapy: from identification to clinical applications. Chin Med J (Engl). 2022;135(11):1285–98.
Cheever MA, Allison JP, Ferris AS, Finn OJ, Hastings BM, Hecht TT, Mellman I, Prindiville SA, Viner JL, Weiner LM, et al. The prioritization of cancer antigens: a national cancer institute pilot project for the acceleration of translational research. Clin Cancer Res. 2009;15(17):5323–37.
Saxena M, van der Burg SH, Melief CJM, Bhardwaj N. Therapeutic cancer vaccines. Nat Rev Cancer. 2021;21(6):360–78.
Linette GP, Carreno BM. On the twentieth anniversary of dendritic cell vaccines - riding the next wave. Cancer Res. 2022;82(6):966–8.
Yu Z, Liu W, He Y, Sun M, Yu J, Jiao X, Han Q, Tang H, Zhang B, Xian Y, et al. HLA-A2.1-restricted ECM1-derived epitope LA through DC cross-activation priming CD8(+) T and NK cells: a novel therapeutic tumour vaccine. J Hematol Oncol. 2021;14(1):71.
Dersh D, Hollý J, Yewdell JW. A few good peptides: MHC class I-based cancer immunosurveillance and immunoevasion. Nat Rev Immunol. 2021;21(2):116–28.
Khawar MB, Sun H. CAR-NK cells: from natural basis to design for kill. Front Immunol. 2021;12:707542.
Poorebrahim M, Mohammadkhani N, Mahmoudi R, Gholizadeh M, Fakhr E, Cid-Arregui A. TCR-like CARs and TCR-CARs targeting neoepitopes: an emerging potential. Cancer Gene Ther. 2021;28(6):581–9.
Leko V, Rosenberg SA. Identifying and targeting human tumor antigens for T cell-based immunotherapy of solid tumors. Cancer Cell. 2020;38(4):454–72.
Niu X, Chen L, Li Y, Hu Z, He F. Ferroptosis, necroptosis, and pyroptosis in the tumor microenvironment: perspectives for immunotherapy of SCLC. Semin Cancer Biol. 2022;86(Pt 3):273–85.
Zhou J, Wang G, Chen Y, Wang H, Hua Y, Cai Z. Immunogenic cell death in cancer therapy: present and emerging inducers. J Cell Mol Med. 2019;23(8):4854–65.
Inoue H, Tsutsumi H, Tanaka K, Iwama E, Shiraishi Y, Hirayama A, Nakanishi T, Ando H, Nakajima M, Shinozaki S, et al. Increased plasma levels of damage-associated molecular patterns during systemic anticancer therapy in patients with advanced lung cancer. Transl Lung Cancer Res. 2021;10(6):2475–86.
Fucikova J, Kepp O, Kasikova L, Petroni G, Yamazaki T, Liu P, Zhao L, Spisek R, Kroemer G, Galluzzi L. Detection of immunogenic cell death and its relevance for cancer therapy. Cell Death Dis. 2020;11(11):1013.
Xie C, Zhou X, Liang C, Li X, Ge M, Chen Y, Yin J, Zhu J, Zhong C. Apatinib triggers autophagic and apoptotic cell death via VEGFR2/STAT3/PD-L1 and ROS/Nrf2/p62 signaling in lung cancer. J Exp Clin Cancer Res. 2021;40(1):266.
Yamamoto K, Venida A, Yano J, Biancur DE, Kakiuchi M, Gupta S, Sohn ASW, Mukhopadhyay S, Lin EY, Parker SJ, et al. Autophagy promotes immune evasion of pancreatic cancer by degrading MHC-I. Nature. 2020;581(7806):100–5.
Tong X, Tang R, Xiao M, Xu J, Wang W, Zhang B, Liu J, Yu X, Shi S. Targeting cell death pathways for cancer therapy: recent developments in necroptosis, pyroptosis, ferroptosis, and cuproptosis research. J Hematol Oncol. 2022;15(1):174.
Yang F, Xiao Y, Ding JH, Jin X, Ma D, Li DQ, Shi JX, Huang W, Wang YP, Jiang YZ, et al. Ferroptosis heterogeneity in triple-negative breast cancer reveals an innovative immunotherapy combination strategy. Cell Metab. 2023;35(1):84-e100108.
Gao W, Wang X, Zhou Y, Wang X, Yu Y. Autophagy, ferroptosis, pyroptosis, and necroptosis in tumor immunotherapy. Signal Transduct Target Ther. 2022;7(1):196.
Morad G, Helmink BA, Sharma P, Wargo JA. Hallmarks of response, resistance, and toxicity to immune checkpoint blockade. Cell. 2021;184(21):5309–37.
Teo MY, Seier K, Ostrovnaya I, Regazzi AM, Kania BE, Moran MM, Cipolla CK, Bluth MJ, Chaim J, Al-Ahmadie H, et al. Alterations in DNA damage response and repair genes as potential marker of clinical benefit from PD-1/PD-L1 blockade in advanced urothelial cancers. J Clin Oncol. 2018;36(17):1685–94.
Yu Y, Wang S, Su N, Pan S, Tu B, Zhao J, Shen Y, Qiu Q, Liu X, Luan J, et al. Increased circulating levels of CRP and IL-6 and decreased frequencies of T and B lymphocyte subsets are associated with immune-related adverse events during combination therapy with PD-1 inhibitors for liver cancer. Front Oncol. 2022;12:906824.
von Itzstein MS, Khan S, Gerber DE. Investigational biomarkers for checkpoint inhibitor Immune-related adverse event prediction and diagnosis. Clin Chem. 2020;66(6):779–93.
Liu X, Shi Y, Zhang D, Zhou Q, Liu J, Chen M, Xu Y, Zhao J, Zhong W, Wang M. Risk factors for immune-related adverse events: what have we learned and what lies ahead? Biomark Res. 2021;9(1):79.
Li S, Mirlekar B, Johnson BM, Brickey WJ, Wrobel JA, Yang N, Song D, Entwistle S, Tan X, Deng M, et al. STING-induced regulatory B cells compromise NK function in cancer immunity. Nature. 2022;610(7931):373–80.
Chen J, Zhao X, Yuan Y, Jing JJ. The expression patterns and the diagnostic/prognostic roles of PTPN family members in digestive tract cancers. Cancer Cell Int. 2020;20:238.
Hao Y, Zhou X, Li Y, Li B, Cheng L. The CD47-SIRPα axis is a promising target for cancer immunotherapies. Int Immunopharmacol. 2023;120:110255.
Boutros C, Tarhini A, Routier E, Lambotte O, Ladurie FL, Carbonnel F, Izzeddine H, Marabelle A, Champiat S, Berdelou A, et al. Safety profiles of anti-CTLA-4 and anti-PD-1 antibodies alone and in combination. Nat Rev Clin Oncol. 2016;13(8):473–86.
Peng D, Fu M, Wang M, Wei Y, Wei X. Targeting TGF-β signal transduction for fibrosis and cancer therapy. Mol Cancer. 2022;21(1):104.
Wang Y, Jia Z, Liang C, He Y, Cong M, Wu Q, Tian P, He D, Miao X, Sun B, et al. MTSS1 curtails lung adenocarcinoma immune evasion by promoting AIP4-mediated PD-L1 monoubiquitination and lysosomal degradation. Cell Discov. 2023;9(1):20.
Yip RKH, Rimes JS, Capaldo BD, Vaillant F, Mouchemore KA, Pal B, Chen Y, Surgenor E, Murphy AJ, Anderson RL, et al. Mammary tumour cells remodel the bone marrow vascular microenvironment to support metastasis. Nat Commun. 2021;12(1):6920.
Bhat AA, Nisar S, Singh M, Ashraf B, Masoodi T, Prasad CP, Sharma A, Maacha S, Karedath T, Hashem S, et al. Cytokine- and chemokine-induced inflammatory colorectal tumor microenvironment: emerging avenue for targeted therapy. Cancer Commun (Lond). 2022;42(8):689–715.
Wei CY, Zhu MX, Zhang PF, Huang XY, Wan JK, Yao XZ, Hu ZT, Chai XQ, Peng R, Yang X, et al. PKCα/ZFP64/CSF1 axis resets the tumor microenvironment and fuels anti-PD1 resistance in hepatocellular carcinoma. J Hepatol. 2022;77(1):163–76.
Fan W, Wang D, Li G, Xu J, Ren C, Sun Z, Wang Z, Ma W, Zhao Z, Bao Z, et al. A novel chemokine-based signature for prediction of prognosis and therapeutic response in glioma. CNS Neurosci Ther. 2022;28(12):2090–103.
Download references
Acknowledgements
Not applicable.
This study was supported by National Natural Science Foundation of China grants (81972484, 82272667), High-level Innovation Team of Nanjing Medical University Program (JX102GSP201727), The Collaborative Innovation Center for Tumor Individualization Program (JX21817902/008), and Beijing Xisike Clinical Oncology Research Foundation (Y-2019AZZD-00680).
Author information
Kai Yang, Rongrong Lu and Jie Mei contributed equally to this work.
Authors and Affiliations
Department of Oncology, The First Affiliated Hospital of Nanjing Medical University, Nanjing, 210029, P. R. China
Kai Yang, Rongrong Lu, Jie Mei, Kai Cao, Tianyu Zeng, Yijia Hua, Xiang Huang, Wei Li & Yongmei Yin
Gusu School, Nanjing Medical University, Nanjing, China
You can also search for this author in PubMed Google Scholar
Contributions
K.Y., R.L. and J.M. wrote the main manuscript text, K.C. and T.Z prepared figures 1-5 and Y.H. prepared tables 1-3. All authors reviewed the manuscript.
Corresponding authors
Correspondence to Xiang Huang , Wei Li or Yongmei Yin .
Ethics declarations
Ethics approval and consent to participate, consent for publication, competing interests.
The authors declare no competing interests.
Additional information
Publisher’s note.
Springer Nature remains neutral with regard to jurisdictional claims in published maps and institutional affiliations.
Rights and permissions
Open Access This article is licensed under a Creative Commons Attribution 4.0 International License, which permits use, sharing, adaptation, distribution and reproduction in any medium or format, as long as you give appropriate credit to the original author(s) and the source, provide a link to the Creative Commons licence, and indicate if changes were made. The images or other third party material in this article are included in the article's Creative Commons licence, unless indicated otherwise in a credit line to the material. If material is not included in the article's Creative Commons licence and your intended use is not permitted by statutory regulation or exceeds the permitted use, you will need to obtain permission directly from the copyright holder. To view a copy of this licence, visit http://creativecommons.org/licenses/by/4.0/ . The Creative Commons Public Domain Dedication waiver ( http://creativecommons.org/publicdomain/zero/1.0/ ) applies to the data made available in this article, unless otherwise stated in a credit line to the data.
Reprints and permissions
About this article
Cite this article.
Yang, K., Lu, R., Mei, J. et al. The war between the immune system and the tumor - using immune biomarkers as tracers. Biomark Res 12 , 51 (2024). https://doi.org/10.1186/s40364-024-00599-5
Download citation
Received : 06 December 2023
Accepted : 10 May 2024
Published : 30 May 2024
DOI : https://doi.org/10.1186/s40364-024-00599-5
Share this article
Anyone you share the following link with will be able to read this content:
Sorry, a shareable link is not currently available for this article.
Provided by the Springer Nature SharedIt content-sharing initiative
- Tumor immunity
- Immune biomarker
- Immunotherapy
Biomarker Research
ISSN: 2050-7771
- General enquiries: [email protected]
Thank you for visiting nature.com. You are using a browser version with limited support for CSS. To obtain the best experience, we recommend you use a more up to date browser (or turn off compatibility mode in Internet Explorer). In the meantime, to ensure continued support, we are displaying the site without styles and JavaScript.
- View all journals
- My Account Login
- Explore content
- About the journal
- Publish with us
- Sign up for alerts
- Open access
- Published: 27 May 2024
Virus-like structures for combination antigen protein mRNA vaccination
- Jingjing Zhang 1 , 2 na1 ,
- Yanmei Li 1 na1 ,
- Fengyuan Zeng 1 ,
- Changyong Mu 1 ,
- Change Liu 1 ,
- Lichun Wang 1 ,
- Xiaowu Peng 1 ,
- Liping He 1 ,
- Yanrui Su 1 ,
- Hongbing Li 1 ,
- An Wang 1 ,
- Lin Feng 1 ,
- Dongxiu Gao 1 ,
- Zhixiao Zhang 1 ,
- Gang Xu 1 ,
- Yixuan Wang 1 ,
- Rong Yue 1 ,
- Junbo Si 1 ,
- Lichun Zheng 1 ,
- Xiong Zhang 1 ,
- Fuyun He 1 ,
- Hongkun Yi 1 ,
- Zhongshu Tang 1 ,
- Gaocan Li 1 ,
- Kaili Ma ORCID: orcid.org/0000-0002-5242-3000 1 , 2 &
- Qihan Li ORCID: orcid.org/0000-0002-8932-4516 1
Nature Nanotechnology ( 2024 ) Cite this article
1904 Accesses
5 Altmetric
Metrics details
- Biomaterials
- Nanobiotechnology
Improved vaccination requires better delivery of antigens and activation of the natural immune response. Here we report a lipid nanoparticle system with the capacity to carry antigens, including mRNA and proteins, which is formed into a virus-like structure by surface decoration with spike proteins, demonstrating application against SARS-CoV-2 variants. The strategy uses S1 protein from Omicron BA.1 on the surface to deliver mRNA of S1 protein from XBB.1. The virus-like particle enables specific augmentation of mRNAs expressed in human respiratory epithelial cells and macrophages via the interaction the surface S1 protein with ACE2 or DC-SIGN receptors. Activation of macrophages and dendritic cells is demonstrated by the same receptor binding. The combination of protein and mRNA increases the antibody response in BALB/c mice compared with mRNA and protein vaccines alone. Our exploration of the mechanism of this robust immunity suggests it might involve cross-presentation to diverse subsets of dendritic cells ranging from activated innate immune signals to adaptive immune signals.
Similar content being viewed by others
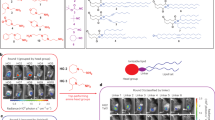
Enhancing the immunogenicity of lipid-nanoparticle mRNA vaccines by adjuvanting the ionizable lipid and the mRNA
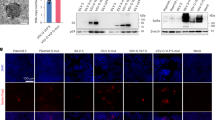
Dendritic-cell-targeting virus-like particles as potent mRNA vaccine carriers
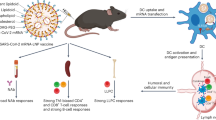
Adjuvant lipidoid-substituted lipid nanoparticles augment the immunogenicity of SARS-CoV-2 mRNA vaccines
Since the global coronavirus disease 2019 (COVID-19) pandemic caused by severe acute respiratory syndrome coronavirus 2 (SARS-CoV-2) infection began at the end of 2019 1 , various vaccines against the disease have been developed and marketed 2 . However, the application of these vaccines did not completely constrain pandemic spread 3 , and the antibody levels found in epidemiological surveys have shown limited duration and low efficacy against viral variants 4 . These findings suggest that the interaction between SARS-CoV-2 and the host immune system is largely unknown, and the development of novel vaccines is needed 5 . Fortunately, breakthroughs in mRNA vaccines based on the successful development of lipid nanodelivery systems have provided new technical possibilities. Lipid nanoparticle (LNP) delivery of mRNA encoding an antigen into cells via fusion of the artificial lipid membrane and cellular plasma membrane results in effective expression of the viral spike protein (S protein) antigen in cells and induces subsequent presentation of the S antigen to activate innate/adaptive immunity via dendritic cells (DCs) and macrophages 6 .
According to viral immunological studies, the structural and/or non-structural antigens produced during viral replication in cells can be recognized by the cellular pattern recognition receptors of the innate immune system in the first event of antigenic signal stimulation of the host immune response. This implies that mRNA has the advantage of eliciting an immune response via such a natural route of immunity 7 ; its strategy is, to some extent, similar to the infectious mechanism of the membranous virus SARS-CoV-2, although live viruses usually utilize the S protein in the viral membrane to bind specifically with the ACE2 receptor in the cellular membrane for viral genome internalization 8 . Therefore, when lipid delivery particles encapsulating mRNA molecules are loaded with S protein on their surface, these lipid particles might possess a virus-like structure (VLS) that enables targeting to cells via the receptor. In particular, the S protein of SARS-CoV-2 was found to interact with ACE2 or DC-SIGN receptors expressed on DC and macrophage surfaces 9 , 10 . These findings lead to the logical inference that if the proposed VLS system could be constructed, it would interact with not only the muscle cells or epithelium of injected local tissue but also the innate immune cells (for example, DCs and macrophages) carrying the ACE2 receptor or DC-SIGN 9 , 10 . Previously, an inhalable nanovaccine with a biomimetic coronavirus structure mimicking viral genetic material encapsulated by liposomes with receptor-binding domains (RBDs) of S protein loaded on the surface as ‘spikes’ to simulate the viral structure was reported, suggesting the possibility of VLS 7 . Therefore, the aim of this study was to design a VLS particle to encapsulate mRNA molecules and load proteins and to investigate its immunological characteristics, especially its capacity to elicit an immune response in a mouse model through surface S1 protein binding specifically to DC-SIGN on DCs and macrophages. The data obtained suggested that this strategy for delivering mRNA specifically into DCs through the VLS structure is very significant in comparison with regular mRNA vaccines.
Characterization of VLS
An important technical basis for the previous development of SARS-CoV-2 mRNA vaccines was the association of mRNA molecules with cationic lipids through static attraction and hydrogen bonding, which led to the encapsulation of mRNA molecules by LNPs 11 . In our VLS design, a lipid carrier encapsulating mRNA was required to load a surface protein, which might require a greater cationic lipid charge capacity for charge complexation in an ionic environment 12 . For lipid particles to acquire a greater capacity to carry mRNA and protein simultaneously, our design included two cationic lipid molecules, ((2-(2-hydroxyethoxyl)ethyl)azanddiyl)bis(hexane-6,1-diyl)bis(2-hexyldecanoate) and 1,2-dioleoyl-3-trimethylammonium propane; one central phospholipid, 1,2-dierucoyl-phosphatidylcholine; and one poly-polyethylene glycol (PEG) molecule, methoxypoly(ethylene glycol)- N -tetradecyltetradecanamide-1-2k. An LNP with this lipid composition and the mRNA encoding the SARS-CoV-2 XBB.1 strain S1 protein was produced through a microfluidic process (Supplementary Fig. 1a ). The structural parameters of these characterized particles (Extended Data Table 1 ) and the identified mRNA molecules associated with the quantification of lipids (Supplementary Fig. 1a ) suggest the typical features of mRNA vaccines. Moreover, this LNP was capable of delivering its encapsulated mRNA into 293 cells in a dose-dependent manner (Fig. 1a,b ). Furthermore, the expressed and purified S1 recombinant protein (Supplementary Fig. 1a ) was loaded on lipid particles encapsulating mRNA for the construction of VLSs with structural characteristics similar to those of mRNA vaccines (Extended Data Table 1 ). Our electron microscopy observations suggested that the loading process was irreversible (Supplementary Fig. 1b ), and the morphological characterization of this VLS revealed particles with some bulges on the surface with differences compared to the LNP-encapsulated mRNA alone (Fig. 1c ). Adding the S antibody enables the flocking of particles by antibody bridges (Fig. 1c ). Structural analysis of the VLSs by immunoprecipitation with a specific S antibody suggested that the precipitated VLS particles contained mRNA molecules and S1 protein (Fig. 1d ). These data support the use of VLS particles in our designed model.

a , Fluorescence micrographs revealed significant GFP expression for LNP, LNP encapsulating GFP mRNA and VLS. Scale bars, 250 μm. b , S1 proteins were assessed by Western blotting analysis of 293T cells transfected with LNPs or VLSs for 48 h. Left: S1 protein expression in 293T cells following transfection of either EGFP or S1 mRNA compared with LNPs; right: S1 protein expression in 293T cells following transfection with VLSs containing different doses of S1 mRNA. GAPDH was included as a protein loading control. The intensities of the signals (above the band) on Western blots were quantified by densitometric analysis with ImageJ (v.1.8.0.112). c , Electron micrographs of LNPs, LNPs encapsulating S1 mRNA, VLS and VLSs treated with a specific anti-S1 antibody. Scale bars, 200 nm. Experiments in a – c were replicated twice. d , Contents of protein (either S1 or N) and mRNA in total sample, penetration and elution. ‘IP-S1’ indicates that the samples were tested following incubation overnight with a specific anti-S1 antibody, and ‘IP-N’ indicates that the samples were incubated with an anti-N antibody. The experimental loading volumes were equal and identified by Western blotting and SDS‒PAGE silver staining (Input). The data are shown as the means ± s.d. from three independent experiments.
VLSs enable cell transfection and target ACE2/DC-SIGN molecules
Compared with the LNPs of the mRNA vaccine, the VLSs improved the delivery capability of the mRNA when the protein was loaded on the surface. Binding to the ACE2 or DC-SIGN molecules of the S1 protein might result in VLSs targeting cells with both receptors in local tissue 12 , 13 . Our work indicated that VLSs were capable of not only transfecting 293 cells with high efficacy (Fig. 2a ) but also of enhancing mRNA expression in cultured 16HBE cells, which showed not only copies of target mRNA (Fig. 2b ) but also the expressed protein (Fig. 2a ) and presented restitution with the addition of antibodies against S or ACE2 (Fig. 2c,d ). The same was true for the THP-1 cells (Fig. 2a,b ). Based on our identified data on approximately 16HBE cells expressing ACE2 and THP-1 cells expressing ACE2/DC-SIGN (Supplementary Fig. 2 ), the enhanced expression of mRNA in both cell lines is probably due to the mediation of ACE2 or DC-SIGN interactions with the S1 protein. Furthermore, treatment of THP-1 cells with a specific antibody against DC-SIGN also interfered with the expression of VLS mRNA (Fig. 2c,d ). This suggested that VLSs enable the delivery of mRNA into human macrophages via S1 through interactions with ACE2 and DC-SIGN receptors, which is consistent with reported data 10 . Furthermore, mouse DCs (JASWIIs) expressing DC-SIGN were found to interact with the S1 protein (Supplementary Fig. 3a ). Transfection of these cells with VLS-encapsulated EGF or S1 mRNA led to obviously greater mRNA expression than transfection with LNP-encapsulated mRNA alone, as the specific blocking of antibodies against S or DC-SIGN eliminated this effect (Fig. 2c,d and Supplementary Fig. 3c ). The same experiment using mouse RAW264.7 macrophages with DC-SIGN also provided similar results (Fig. 2c and Supplementary Fig. 3b ). Based on previously confirmed data showing that human DCs and macrophages were infected by SARS-CoV-2 due to their expression of ACE2/DC-SIGN 14 , 15 , our results suggested that the interaction of the VLS with ACE2 or DC-SIGN receptors might be involved in the activation of innate immunity.

a , S1 proteins were expressed by Western blotting for different cells. c , S1 proteins were expressed by Western blotting which used antibodies to block receptors for different cells or S1 (N) protein for VLSs. LNPs and VLSs encapsulating S1 mRNA were incubated with 293T cells, 16HBE cells, THP-1 cells, DCs and RAW264.7 cells. Experiments in a and c were repeated twice. b , mRNA copies transfected into different cells were assessed by qRT-PCR. d , mRNA copies transfected into cells were assessed by qRT‒PCR which used antibodies to block receptors for cells or S1 (N) protein for VLSs. b , d, n = 3 independent samples, mean ± s.d. The blue text in the yellow frames shows the different antibody-treated samples or cells. Significant differences were determined using one-way analysis of variance (ANOVA) with Tukey’s post hoc test.
The interaction of VLSs with DCs and macrophages
Previous data suggested that the intense transcriptional response induced by LNPs to mRNA vaccines in cells was due to exogenous injury induced by LNPs and was thought to be an adverse reaction to mRNA vaccines 16 , 17 , 18 , 19 . We investigated whether a decrease in the activity of our VLS could be achieved through the binding of the S1 protein to the receptors. In our study, the expression of some molecules with immunological activation in innate/adaptive immunity tended to increase in 16HBE cells treated with VLSs (Fig. 3a ), and increased expression of immune regulators and decreased expression of inflammatory mediators, such as tumour necrosis factor (TNF), were detected in the THP-1 cells (Fig. 3b ). In addition, the expression of molecules, including TNF and GATA-3, tended to decrease in the JAWSII strain of mouse DCs, while the expression of members of the interferon (IFN) family tended to increase (Fig. 3c ). A mild and balanced upregulation of the transcription profile of immune regulators and inflammatory factors was observed in RAW264.7 mouse macrophages (Fig. 3d ). Interestingly, the upregulated expression of members of the interferon family in epithelial cells was similar to that in DCs and macrophages (Fig. 3a,c ). These results suggest a certain immune activation role of VLSs in innate immunity that is different from that of mRNA/LNPs and support our hypothesis that the effect of the S1 protein on the VLS surface could lead to not only mRNA delivery but also activation of the DC-SIGN signalling pathway in DCs.
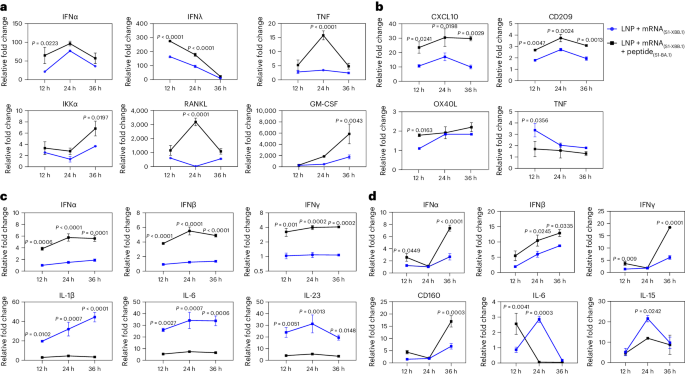
a – d , Expression profiles of some major genes associated with the immune response in 16HBE ( a ), THP-1 ( b ), JASWII DC ( c ) and RAW264.7 ( d ) cells transfected with LNPs encapsulating only S1 mRNA or both S1 mRNA and S1 protein at 24 h after transfection. The blue represents LNPs encapsulating only S1 mRNA, and the black represents VLSs. The data are shown as the means ± s.d. from three independent experiments ( n = 3). Significant differences were determined using one-way ANOVA with Tukey’s post hoc test.
Immunization of mice with VLSs elicits a discrepancy in immune signal transduction
Our investigation of the interaction between VLSs and the immune system suggested that the mRNA encapsulated in the VLS, whether encoding GFP or the S1 protein, was expressed dynamically in local tissue (Supplementary Fig. 4 ), similar to what has been reported for mRNA vaccines 20 . Interestingly, the rate of S1 antigen colocalization with DCs or macrophages in local tissue in mice in the VLS group was greater than that in mice in the mRNA- or protein-alone groups (Fig. 4a and Supplementary Fig. 5a,b ). The ratio of CD86 + DCs to CD83+ DCs, which suggested the maturity of DCs stimulated by toll-like receptor (TLR) signals, was greater in the spleens of mice in the VLS group on days 3–7 after primary immunization than in those in the mRNA or protein groups (Fig. 4b ). The ratio of specific CD4 + to CD8 + memory T cells in the spleen was also greater in the boosted VLS group and in the group that received DCs pretreated with VLS than in the other two groups (Fig. 4c,d ), which suggested that the interaction of VLSs with mouse DCs via S1 protein binding specifically to the DC-SIGN receptor followed by the effective expression of mRNA to augment the activation efficiency of DCs followed by subsequent activation of T cells was significant. Further antibody measurements revealed a greater antibody titre in mice that received DCs pretreated with VLSs on day 21 after injection than in mice that received DCs pretreated with mRNA or protein vaccines (Fig. 4e ). Subsequent analysis of the transcription levels of various important immune molecules in the local tissue of mice injected with the experimental VLS vaccine between 24 and 48 h after immunization suggested increased expression of some functional molecules related directly to DCs and macrophage activation, such as CXCL10 and MHCI, compared with the expression found in mice immunized with mRNA or protein alone (Fig. 4f ). The thousand-fold increase in CXCL10 suggested that the DCs activated by VLSs could be classified as cDCs 21 . Further Kyoto Encyclopedia of Genes and Genomes orthology transcriptomic analysis of lymphocytes collected from the spleens of immunized mice at various time points after immunization revealed a characteristic dynamic profile in the VLS group in comparison to that in the mRNA and protein groups (Fig. 4g ). These variations in all transcriptional profiles suggested a discrepancy in host immune responses in individuals immunized with VLSs and mRNA or protein.
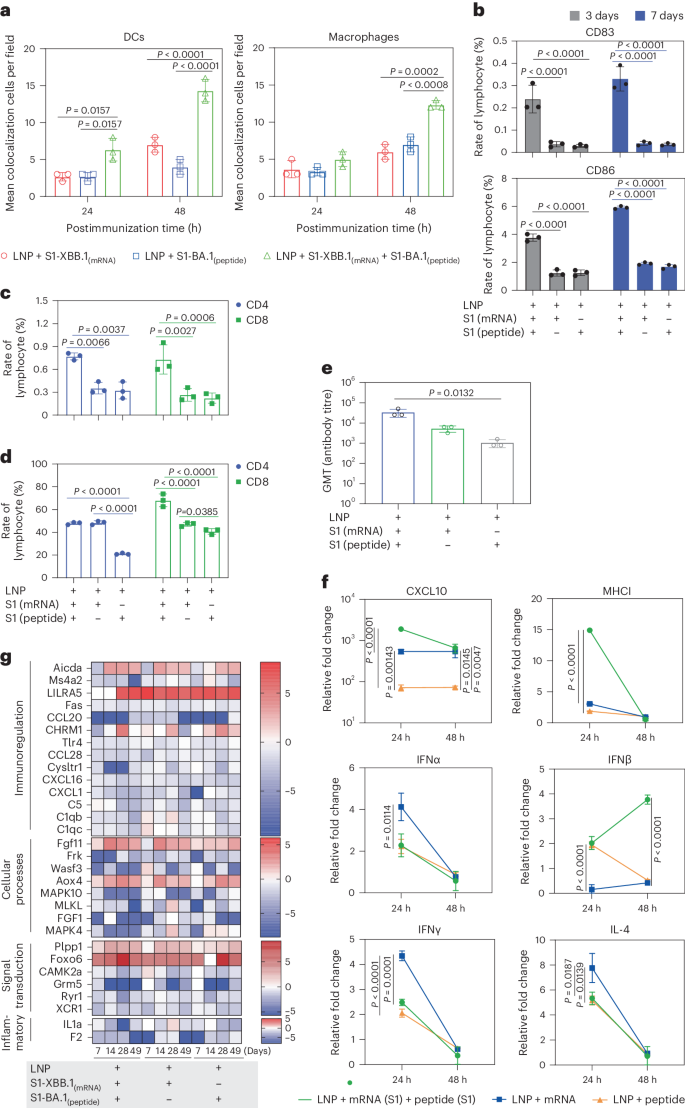
a , Representative colocalization rates of S1 expression and DCs/macrophages in muscles of mice injected i.m. with VLSs, LNPs encapsulating S1 peptide or LNPs encapsulating S1 mRNA at 24 and 48 h. b , Ratio of CD86 + to CD83 + DCs in the spleens of mice after immunization with VLSs, LNPs encapsulating S1 peptide or LNPs encapsulating S1 mRNA for 3 days and 7 days. c , d , Ratio of specific memory T cells ( c ) and activated T cells ( d ) with marker molecules of CD8 in the spleen of the boosted and DC-treated transfusion VLSs, LNPs encapsulating S1 peptide or LNPs encapsulating S1 mRNA. e , Antibody titre in mice on day 21 after the transfusion of DCs from different treatment groups. f , Expression profiles at 24 and 48 h of some major genes associated with inflammation and the innate immune response in the muscle tissue of mice injected i.m. with VLSs, LNPs encapsulating S1 peptide or LNPs encapsulating S1 mRNA. g , Expression profiles of major genes associated with the activation or differentiation of immunoregulation, cellular processes, signal transduction and inflammation in lymph nodes at 7, 14 and 28 days after primary immunization and 21 days after booster immunization. The colour bars represent the log 2 (fold change) compared with the value in the LNP group. The redder the colour, the more obviously the gene transcription level is upregulated. The relative expression levels of inflammatory cytokines in tissue were normalized to their levels in the blank control group (LNP) by using the comparative Ct (ΔΔCt) method. The data are shown as the means ± s.d. from three independent experiments ( n = 3). Significant differences were determined using one-way ANOVA with Tukey’s post hoc test.
VLSs elicit a more robust immune response in mice
Based on the analysis of differences between our VLS vaccine and the mRNA vaccine (phase III clinical trial) currently used in the population (over 18 years old) (Supplementary Fig. 6 ), our immunological data suggested that the VLSs with mRNA and protein contents equal to those of the mRNA or protein vaccine elicited a robust immune response in immunized BALB/c mice, which had a much greater level of binding antibodies against the S antigen of the Omicron strain after immunization than that of mice immunized with mRNA or protein alone; the high level was maintained for more than eight months (Fig. 5a ), and the mice showed obvious reactivity against S antigens of the Wuhan, Beta and Delta strains, with slightly lower levels (Fig. 5b ). The synchronous detection of neutralizing antibodies against the variants with a pseudovirus and real virus also revealed similar results (Fig. 5c ), in which neutralizing antibodies against the variants reached a level higher than 1:10 3 , which was confirmed to be effective for immune protection in previous studies of animals and humans 15 , 16 . The levels of antibodies against the RBD of the Wuhan, Omicron and XBB.1 strains were greater in the VLS group than in the other two groups (Fig. 5d ). Compared with those in the other two groups, the levels of IgA secreted in the upper respiratory tract and in the serum were increased post-VLS immunization, especially after booster immunization, in the mice in the VLS group (Fig. 5e ). Furthermore, the comparison of the ratio of activated B cells in the lymph nodes of mice in the VLS and mRNA groups indicated that the ratio of activated B cells in the VLS group was greater than that in the mRNA group (Fig. 5f ). Importantly, an ELISpot assay specific for IFNγ and interleukin-4 (IL-4) showed a more intensive T-cell response in the VLS group than in the mRNA and protein groups on day 90 after immunization (Fig. 5g ). Due to the obvious intensification of the IL-4 response observed, multiple cytokines in the sera of these mice were assayed at the same time, and the results indicated lower levels of most cytokines in the VLS group than in the mRNA or protein group (Fig. 5h ). All the data suggested that the immune response elicited by VLSs should differ from that elicited by mRNAs or proteins based on the known activating effect of VLSs on innate immunity.

a , b , SARS-CoV-2 titres of specific antibodies for Omicron ( a ) and wild-type (WT), Beta and Delta ( b ) strains whose production was induced by VLSs, LNPs encapsulating S1 peptide or LNPs encapsulating S1 mRNA in mice. The samples were obtained on days 35, 49, 120, 180 and 240 after primary injection. c , Titres of neutralizing antibodies (NAb) against the Omicron real virus strain BA.5 and the pseudovirus whose production was induced by VLSs, LNPs encapsulating S1 peptide or LNPs encapsulating S1 mRNA in mice. d , Titres of IgG antibodies against the RBD of the Omicron strain, XBB.1 strain and WT strain whose production was induced by VLSs, LNPs encapsulating S1 peptide or LNPs encapsulating S1 mRNA in mice. e , Titres of IgA antibodies against the spike protein of the Omicron strain whose production was induced by VLSs, LNPs encapsulating S1 peptide or LNPs encapsulating S1 mRNA in mouse BALF and serum 21 and 49 days after immunization. f , The activated B cells observed in lymph nodes of mice treated by VLS and mRNA vaccine. g , ELISpot responses of IFNγ and IL-4 in mice injected i.m. with VLSs, LNPs encapsulating S1 peptide or LNPs encapsulating S1 mRNA on day 49 after primary immunization. The orange frame represents LNPs encapsulating 5, 10, 20 and 40 μg S1 mRNA, from light to dark; the blue border represents 5, 10, 20 and 30 μg S1 peptide; and the orange frame in the blue border from light to dark represents LNPs encapsulating different combinations of RNA and peptide at different doses. SFC, spot-forming cells. h , Cytokines in the sera of mice immunized with VLSs, LNPs encapsulating S1 peptide or LNPs encapsulating S1 mRNA on day 28 after booster immunization. All assay values were calculated by subtracting the values detected with blank mouse serum. The data are shown as the means ± s.d. from three independent experiments ( n = 3). Significant differences were determined using one-way ANOVA with Tukey’s post hoc test.
Effective immune protection elicited in ACE +/+ mice immunized with VLSs
In our viral challenge test involving both ACE +/+ mice and hamsters (Supplementary Fig. 7 ), the results indicated that the VLSs completely protected the challenged mice from the Omicron BA.5 strain, XBB.1 and Wuhan strains because no symptoms were observed (Extended Data Table 2 ). Very little or no viral replication was detected in various organs of the mice during the infection period (Fig. 6a,b and Supplementary Figs. 8 – 10 ), and no histopathological changes were detected (Extended Data Table 3 ). Significantly, no live virus was detected in the plaque assay in any of the main organs of VLS-immunized mice challenged with XBB.1 in comparison to those of VLS-negative mice (Fig. 6c ). In addition, the transcriptomic profiles of lymphocytes from BA.5-challenged mice were analysed on day 3. The results suggested that the differentially expressed genes related to the functional response of the immune system in the VLS group were obviously different from those in the other two groups (Fig. 6d ). The categorization of B cells, CD4 + cells and CD8 + cells suggested that a greater percentage of DEGs were concentrated in the CD4 + cell and B-cell populations (Fig. 6d ). These genes were mainly transcription-regulating molecules related to lymphocyte proliferation. Combined with the excellent immune protection efficacy in ACE +/+ mice in the VLS group, this unique transcriptional profile suggested an immunological outcome elicited by the integrative effect of diverse signals based on systematic activation of DCs under VLS stimulation through the robust binding of VLSs to DCs.
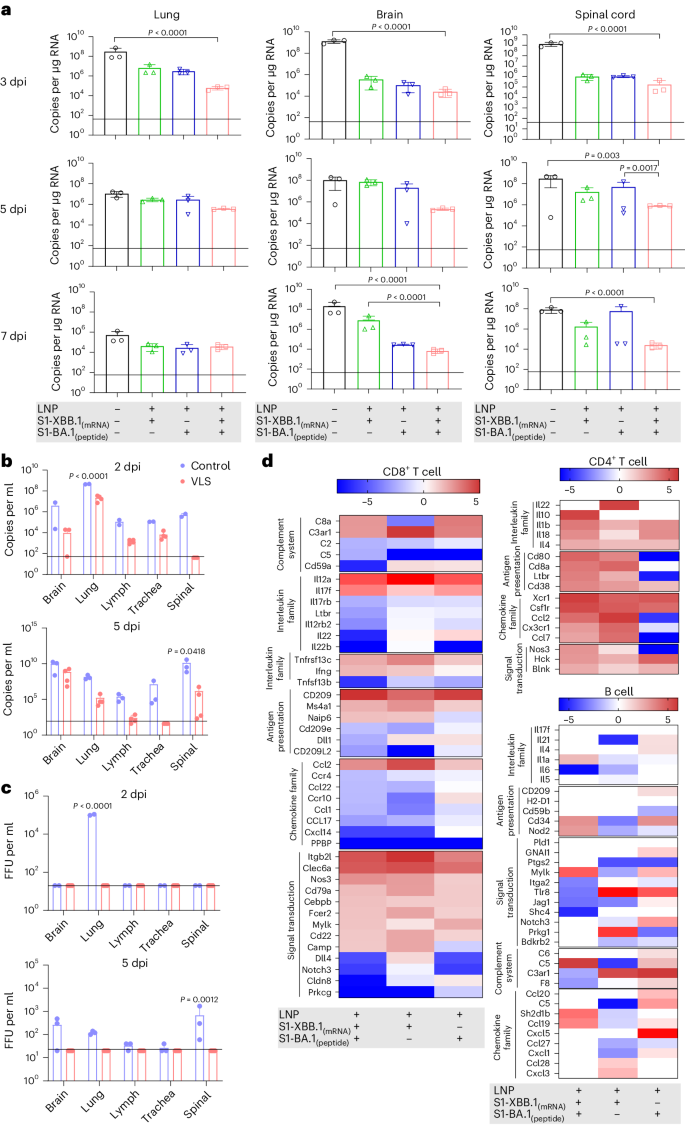
a , The viral loads in the lung, brain and spinal cord of hACE +/+ mice injected i.m. with VLS, LNPs encapsulating S1 peptide, or LNPs encapsulating S1 mRNA were evaluated following infection with the Omicron BA.5 strain, as determined by RT–qPCR. b , c , The viral loads in the brain, lung, lymph, trachea and spinal cord of hACE +/+ mice injected i.m. with VLSs and LNPs following infection with the Omicron XBB.1 strain were evaluated by RT–qPCR ( b ) and the viral titre was determined by virus plaque formation ( c ). Baseline was defined on the basis of the value detected in negative uninfected mice. FFU, focus forming unit; dpi, day(s) postinfection. d , Significantly differentially expressed genes associated with the activation or differentiation of CD8 + T, CD4 + T and B cells isolated from the lymph node tissue of mice injected i.m. with VLSs, LNPs encapsulating S1 peptide or LNPs encapsulating S1 mRNA 3 days after infection with the Omicron BA.5 strain. The colour bars represent the log 2 (fold change) compared with the LNP group. A redder colour indicates more obvious upregulation of the gene transcription level. The data are shown as the means ± s.d. from three independent experiments ( n = 3). Significant differences were determined using one-way ANOVA with Tukey’s post hoc test.
During the past years of the COVID-19 pandemic, mRNA vaccines have provided us with novel vaccine research techniques and novel ideas for vaccine design and development 22 , 23 , 24 . This technological approach induces biological mimicry of infectious agents and offers new possibilities for the development of next-generation COVID-19 vaccines 25 . Based on this progress, our work established an improved lipid system that broadens the capacity of antigen carriage to include mRNA and protein. This system depends on the structure, which consists of mRNA and viral protein combined via specialized lipid particles assembled from two cationic lipids, one central phospholipid and one poly-PEG molecule. This lipid system was capable of efficiently encapsulating mRNA encoding the S1 antigen inside and carrying the S1 protein on its surface. Furthermore, our results revealed that the S1 protein on the surface enables binding with ACE2/DC-SIGN receptors to facilitate the entry of mRNA into cells and increase antigen expression, except for its expression via LNP fusion to the cell membrane. Therefore, we further showed that the S1 protein on the VLS surface plays an important role in guiding mRNA/LNPs to target DCs and macrophages, which results in significantly increased antigen uptake by the immune system. Further analysis of the transcription profiles of these cells suggested that the general trend in which VLSs were able to elicit the expression of multiple immune regulators and inflammatory mediators in a balanced way differed from that of mRNA/LNPs, which implied the significance of the VLS as a vaccine immunogen. This design was further verified by immunological analyses in vivo; we observed greater colocalization of S1 antigen and DCs or macrophages in local tissues and a greater percentage of activated DC populations in the lymph organs of individuals immunized with VLSs than in those immunized with mRNA/LNPs. Further investigation using BALB/c mice that received DCs pretreated with VLSs in vitro revealed a greater ratio of activated T cells in the VLS group than in the mRNA vaccine group. Importantly, mice immunized with the VLSs exhibited a more intense antibody response and stronger cytotoxic T-lymphocyte response than those immunized with the mRNA/LNPs. Interestingly, specific IgA secreted from the upper respiratory tract and in the serum was detected. Based on further protective animal tests, we found that such enhanced immunity provided more effective protection against Omicron BA.5, XBB.1 or the Wuhan strain. Further investigation revealed differences in the systemic upregulation of most genes in the CD4 + cell population and the upregulation of more than 50% of the genes in the B-cell and CD8 + -cell populations in ACE +/+ mice in the VLS group upon challenge. These findings suggested a characteristic systematic immune response in mice immunized with the VLS, possibly because of the integrated effect of diverse signals from the innate immune system. These data support our speculation that VLSs enable interactions with various cells, which leads to not only endogenous antigen protein expression by mRNA and mRNA molecules recognized by intracellular TLRs 26 but also the recognition of exogenous S1 protein by other TLRs in the plasma membrane of diverse subsets of DCs 27 , similar to binding to DC-SIGN or ACE2. Moreover, the antigenic chemotactic effect of epithelial cells transfected with VLSs via lipid fusion enabled the activation of DCs for antigen uptake and generated a certain antigenic signal. These events elicited during VLS immunization might lead to antigenic cross-presentation of diverse subsets of DCs and macrophages to T and B cells and could result in a robust immune response to VLS immunization (Extended Data Fig. 1 ). Therefore, we hypothesized that a strategy based on the specific induction of mRNA expression in DCs and macrophages could facilitate the development of virus-like vaccine candidates enabling the elicitation of an integrative immune response to provide more effective protection against viral variants than the current COVID-19 mRNA vaccine. However, this study has insufficient data to explain the physicochemical structure of the VLSs and the mechanisms underlying their interaction with cells (specifically DCs or macrophages), the signals elicited in these cells and the systematic immunological process in VLS-immunized individuals, which deserves further in-depth investigation.
Ethics statement
Four- to five-week-old K18- hACE2 C57BL/6 mice (human ACE2 gene knock-in C57BL/6 mice) by Cyagen Biosciences (animal license number SCXK (Su) 2022-0016). BALB/c mice and seven- to eight-week-old golden hamsters were purchased from Vital River (animal license numbers SCXK (Jing) 2021-0006 and SCXK (Jing) 2021-0011). All animals were bred in specific pathogen-fee barrier facilities (laboratory license number SYXK (Lu) 2020-0019). The laboratory animals were cared for and used following the ‘3 Rs’ principle and animal welfare guidelines. The animal experiment process and animal-related care and welfare were reviewed and approved by the Animal Experiment Ethics Committee of Shandong WeigaoLitong Biological Products (approval number LACUC-RD3-2022-006).
HEK-293T and 16HBE cells were cultured in Dulbecco’s modified Eagle’s medium (DMEM, Thermo Fisher Scientific) supplemented with 10% fetal bovine serum (FBS; HyClone, GE Healthcare), 10% 100 U ml −1 penicillin and 100 mg ml −1 streptomycin. JASWII dendritic cells were cultured in MEM Alpha (Thermo Fisher Scientific) supplemented with 20% FBS and 5 ng ml −1 murine granulocyte–macrophage colony-stimulating factor (HY-P7361, MCE). RAW264.7 cells were cultured in minimum Eagle’s medium (MEM; Thermo Fisher Scientific) supplemented with 10% FBS. THP-1 cells were cultured in RPMI 1640 (Thermo Fisher Scientific) supplemented with 10% FBS and 0.05 mM β-mercaptoethanol (M917637, Macklin). All cells were purchased from ATCC and maintained at 37 °C with 5% CO 2 .
The novel coronavirus H strain (Omicron BA.5) was isolated from COVID-19 patients in December 2022. The complete genome sequence was uploaded to NCBI GenBank (OQ179919.1). The novel coronavirus KMS-2 strain (Wuhan) was isolated from COVID-19 patients at the Hospital for Infectious Diseases in Yunnan Province in February 2020.
Animal experimental design
C57BL/6-ACE2 (BALB/c or golden hamster) mice were randomly divided into five groups (Supplementary Fig. 7 ).
In group A (immune experimental group, n = 35), C57BL/6-hACE2 mice were divided into four groups: control, VLS, mRNA and peptide. The VLS contained 20 μg of mRNA and 10 μg of protein; the mice in the mRNA group were immunized with 20 μg of mRNA, and the mice in the peptide group were immunized with 10 μg of protein. All the mice underwent booster immunization on the 21st day after primary immunization. At 24, 48 and 72 h after primary immunization, tissues from the immune site were obtained for immunofluorescence detection for qRT–PCR measurement of cytokine expression. Moreover, B, CD4 + T and CD8 + T cells were sorted from lymph nodes at 3, 7 and 14 days after primary immunization and 28 days after booster immunization for transcriptome sequencing.
In group B (immune experimental group, n = 36), C57BL/6-hACE2 mice were divided into three groups: the control, VLS and mRNA groups. The mRNA used for the VLS and mRNA vaccines to explore the tissue site of mRNA molecules with the delivery system. The heart, liver, spleen, kidneys, lungs, brain and lymph nodes were collected at 1, 3, 5 and 7 days after i.m. injection for tissue immunofluorescence detection.
In group C (immune experimental group, n = 72), BALB/c mice were divided into 12 groups: N1–N12. N1–N4 were the mRNA vaccine groups, N5–N8 were the VLS vaccine groups and N9–N12 were the peptide vaccine groups. N1–N4 contained 5, 10, 20 and 40 μg mRNA, respectively. N5–N8 contained 5 μg mRNA and 5 μg peptide, 10 μg mRNA and 5 μg peptide, 10 μg mRNA and 10 μg peptide, and 20 μg mRNA and 10 μg peptide, respectively. N9–N12 contained 5 μg, 10 μg, 20 μg and 30 μg of peptide, respectively. All the mice underwent booster immunization on the 21st day after primary immunization. Blood samples were collected from the tail vein on days 35, 49, 120, 180 and 240 after immunization for antibody detection. At 28 days after booster immunization, blood was collected for neutralizing antibody and binding antibody testing, and the spleen was subjected to lymphocyte separation and ELISpot assays. At the same time, the immune titre of the SARS-CoV-2 vaccine was compared.
In group D (immunoprotective experimental group; C57BL/6-hACE2, n = 75; golden hamster, n = 40), at 28 days after booster immunization, the mice in the control, VLS, mRNA and peptide groups were challenged with SARS-CoV-2 Omicron BA.5 (10 4.5 50% cell culture infectious dose (CCID 50 )), SARS-CoV-2 wild-type (10 4 CCID 50 ) or SARS-CoV-2 Omicron XBB.1 (10 4.5 CCID 50 ) via the intranasal route. The heart, liver, spleen, kidneys, lungs, trachea, brain, spinal cord, lymph nodes and sex organs were collected at 3, 5 and 7 days after viral infection for viral load quantification. Meanwhile, B, CD4 + T and CD8 + T cells were sorted from C57BL/6-hACE2 mouse lymph nodes at 3 days after challenge for transcriptome sequencing.
In group E (transfusion experiment, n = 27), DCs were treated with LNPs-peptide, LNPs-mRNA and VLSs for 12 h. The in vitro-treated DCs (3 × 10 5 cells per mouse) were transfused into mice through the tail vein. The spleen and inguinal lymph nodes were obtained at 3 and 5 days after adoptive transfer for flow cytometry and qRT‒PCR. Antibody titres were measured 21 days after transfusion. The above animal challenge experiments were commissioned by Wuhan Institute of Biological Products.
Synthesis and formulation of the LNP delivery system
According to the molecular weights of ((2-(2-hydroxyethoxyl)ethyl)azanddiyl)bis(hexane-6,1-diyl)bis(2-hexyldecanoate), 1,2-dioleoyl-3-trimethylammonium propane, 1,2-dierucoyl-phosphatidylcholine and methoxypoly(ethylene glycol)- N -tetradecyltetradecanamide-1-2k, these were dissolved in absolute ethanol (Grade of guaranteed reagent) to molecular concentrations of 20%, 10%, 20% and 10%, respectively. All four components were mixed at a molar ratio of 30.68:6.84:15.2:1 in ethanol to prepare the LNP delivery system. The mRNA was diluted according to the requested ratio in buffer of 20 mM sodium acetate, 2.5 mM KCl and 0.1% trehalose. The LNP–mRNA was prepared with NanoAssemblr Ignite+ LNPs (Precision Nanosystems) by mixing blank LNPs with mRNA at a weight ratio of 1:3. The lipid particles were further characterized by Zetasizer Ultra spectrometry.
Synthesis of SARS-CoV-2 S1 mRNA
The S1 sequence of SARS-CoV-2 was amplified and inserted into the PVAX vector by PCR technology. The 5′-untranslated region (UTR) of yellow fever virus was amplified and inserted before the Kozak sequence and the 3′-UTR of the human mitochondrial ribosome was amplified and inserted after the S1 sequence as the 5′-UTR and 3′-UTR of the S1 sequence. The constructed plasmid was linearized with BamHI (catalogue number R0136V, NEB) at 37 °C for 3 h for the in vitro transcription reaction. N1mψ-modified S1 mRNA was synthesized through an in vitro transcription reaction (HiScribe T7 ARCA mRNA Kit, catalogue number E2060S, NEB). The reaction mixture was treated with DNase I and purified using lithium chloride precipitation.
Preparation of the VLS vaccine
The VLS vaccine was prepared by adding LNP–mRNA and peptide to the buffer (0.1% trehalose and 3.5% glucose) and mixing at room temperature for 30 min at 30 r.p.m. before rotating the mixture at 10 r.p.m. at 4 °C overnight. The VLS vaccine was further characterized by Zetasizer Ultra spectrometry.
LNP mRNA- and VLS-transfected cells
Then, 0.5, 1, 2, 5 and 10 μg of mRNA was transfected into 293T cells with the LNP delivery system, and western blot detection was performed at 24 h after transfection. Meanwhile, the mRNA and VLSs were transfected into 16HBE, JASWII DCs, THP-1 cells and RAW264.7 cells for Western blot analysis and qRT‒PCR measurement at 24 h.
Co-immunoprecipitation
Anti-S1 and anti-N antibodies were added to the LNP-encapsulated mRNA and VLS vaccine (the VLS vaccines were loaded with BA.1 S1 protein or N protein). Then, 50 μl of magnetic beads (BeaverBeads Protein A Matrix Antibody Purification Kit, catalogue number 20102, Suzhou) were added and incubated for 30 min at room temperature at 30 r.p.m. Next, the samples with magnetic beads were adsorbed at room temperature for 2–3 min at the magnetic pole, and the supernatant was used for mRNA and protein content measurement. Then, washing buffer was used to wash the beads, and elution buffer was added for elution and measurement of mRNA by qRT‒PCR and protein content by ELISA.
The amount of mRNA in the VLS and mRNA vaccines was measured by qRT‒PCR. Based on the guidelines, the binding site designed for the primer was located in the SARS-CoV-2 S1 gene region, and part of the S1 gene was constructed in the pUC57 plasmid. In vitro-transcribed mRNA was used as a standard sample. The primers were F: TGGATCTGGAGGGAAAGCAGGGCAACT and R: CCGATTGGCAGATCCACCAGAGGTTC, and the TaqMan probe (Sangon Biotech) had the sequence 5′-6FAM-ATGGCTACTTCAAGATCTATAGCAAGC-TAMRA-3′.
The viral loads in the tissues were determined by qPCR with absolute quantification. Based on World Health Organization guidelines, the binding site designed for the primer was located in the SARS-CoV-2 N gene region, and the N gene was constructed on the pUC57 plasmid. The primers were F: GAATGGCTGGCAATGGCGGTGATGCT and R: TTGTTGGCCTTTACCAGACATTTTGCT, and the TaqMan probe (Sangon Biotech) had the sequence 5′-6FAM-TTGCTGCTGCTTGACAGATT-TAMRA-3′. Viral genomic RNA was extracted from tissues using RNAiso Plus (T9108, Takara), and the reactions were performed using a One Step PrimeScript RT‒PCR Kit (Perfect Real Time) (TaKaRa, code RR064A).
For the relative expression of cytokines in tissues and cells, total RNA was extracted with RNAiso Plus (T9108, Takara). Gene expression was expressed as the fold change (2 −∆∆Ct ) relative to the levels in samples from LNP-injected mice or virus-uninfected cells, which were used for calibration. The reactions were performed by using a One-Step SYBR PrimeScript PLUS RT–PCR Kit (TakaRa, code RR096A). The specific primers used are listed in Extended Data Tables 4 and 5 .
Immunofluorescence and confocal microscopy
Muscle tissues were collected and immediately frozen in liquid nitrogen. The tissue sections were embedded in OCT (Tissue-Tek OCT Compound 4583, Sakura) and sliced on a cryostat to a thickness of 5 µm (CM1850, Leica). Tissue sections were fixed and blocked with 5% BSA. For detection of the viral antigen, the sections were sequentially incubated with a primary mouse anti-SARS-CoV-2 S antibody (Sino Biological, catalogue number 40150-V08B1) and an Alexa Fluor 647-conjugated goat anti-mouse IgG secondary antibody (Invitrogen). DCs were detected using an anti-CD11c antibody (Abcam, catalogue number ab33483-N418), and macrophages were detected using an anti-F4/80 antibody (Zhengneng, catalogue number 263101-31B1), an Alexa Fluor 488-conjugated goat anti-rabbit IgG secondary antibody and a 647-conjugated goat anti-mouse IgG secondary antibody (Invitrogen). All cell nuclei were detected using 4,6-diamidino-2-phenylindole and analysed using a confocal microscope (TCS SP2, Leica).
Virus titration
The virus titre was determined with a plaque assay in accordance with standard protocols, as described previously 28 . In brief, the virus was subjected to gradient dilution and added to six-well plates. Carboxymethylcellulose was used as the matrix in a liquid overlay, crystal violet was used as the stain to enhance plaque visualization, and the cells were cultured at 37 °C with 5% CO 2 for 7 days.
Neutralization assay
Briefly, serum was inactivated for 30 min at 56 °C, continuously diluted from 1:4 and mixed with virus at a titre of 100 times the CCID 50 /100 μl and incubated at 37 °C for 2 h. The mixture was added to a six-well plate and used carboxymethylcelluloseas the matrix, and crystal violet was used as the stain to enhance plaque visualization after culture at 37 °C with 5% CO 2 . Meanwhile, the serum neutralization titres of pseudoviruses were measured, and the 50% inhibitory dilution (EC 50 ) was defined as the serum dilution at which the relative light units were reduced by 50% compared with those of the virus control wells (virus + cells) after subtraction of the background relative light units in the control groups with cells only. In brief, pseudovirus (Acro, catalogue number PSSO-HLC016) was incubated with serial dilutions of the test samples continuously diluted from 1:64 in duplicate for 1 h at 37 °C, together with the virus control and cell control wells. Then, 293T-ACE2 cells were added to each well. Following 48 h of incubation in a 5% CO 2 environment at 37 °C, the luminescence was measured.
IFNγ-specific and IL-4-specific ELISpot assay
For the ELISpot assay, mouse IFNγ and IL-4 ELISpot kits (Mabtech) were used according to the manufacturer’s protocol. Briefly, a plate was conditioned and seeded with splenic lymphocytes prior to the addition of 3 μg of stimulant (XBB Spike RBD Protein, catalogue number 40592-V08H144, Sino Biological; B.1.1.529 Spike RBD Protein, catalogue number SPD-C522e, Acro; BA.4/BA.5 Spike RBD Protein, catalogue number SPD-C522R, Acro; BA.2.12.1 Spike RBD Protein, catalogue number SPD-C522q, Acro; BQ.1.1 Spike RBD Protein, catalogue number SPD-C5240, Acro; BA.2.75.2 Spike RBD Protein, catalogue number SPD-C522z, Acro). Then, the cells were added and incubated at 37 °C for 30 h. Next, the cells and medium were removed, and the plate was developed. The coloured spots were counted using an ELISpot reader (Mabtech).
Isolation of B, CD4 + T and CD8a + T cells from mouse lymph nodes
Lymph nodes were isolated under sterile conditions and gently ground, and lymphocytes were separated into a suspension.
B cells were enriched with MajoSort Mouse CD19 Nanobeads (catalogue number SPD-C522R, Acro 480002, BioLegend), CD4 + T cells were enriched with the EasySep Mouse CD4 + T-cell isolation kit (catalogue number SPD-C522R, Acro 19852A, Stemcell), and CD8 + T cells were enriched with the EasySep Mouse CD8a + selection kit (catalogue number SPD-C522R, Acro 18953, Stemcell).
Transcriptome analysis of B, CD4 + T and CD8a + T cells
The B, CD4 + T and CD8a + T cells were sorted by lymph nodes. Total RNA was extracted using TRIzol (catalogue number DP421, Tiangen). RNA quantity and integrity were evaluated using a NanoDrop system and a Bioanalyzer, and the samples were prepared according to Illumina’s instructions and sequenced (Gene Denovo Biotechnology). Genes with 2-fold or greater changes in expression at P < 0.05 in the Kyoto Encyclopedia of Genes and Genomes analyses were selected and grouped into functional categories. All transcriptome sequencing results were uploaded to GSA; the assigned accession number of the submission was CRA010542 .
The S1 antigen was quantified using a SARS-CoV-2 Spike Protein ELISA Kit (Acro, catalogue number RAS-A039), and the nucleoprotein was quantified using a SARS-CoV-2 (2019-nCoV) Nucleoprotein ELISA Kit (Jiya Biotechnology,). S1-RBD IgG assays were performed using SARS-CoV-2 RBD (Wild-Type) Antibody (IgG) Detection Kits (Vazyme, catalogue number DD3201-01), SARS-CoV-2 RBD (Omicron BA.4/5) Antibody (IgG) Detection Kits (Vazyme, catalogue number DD3214-01, China) and Mouse Anti-2019-nCoV (S) IgA Elisa Kits (FineTest, catalogue number 1906).
The S1 antibody assays were performed using a Mouse Anti-SARS-CoV-2 (B.1.1.529) Antibody IgG Titer Serologic Assay Kit (Spike S1) (Acro, catalogue number RAS-T061), Mouse Anti-SARS-CoV-2 Antibody IgG Titer Serologic Assay Kit (Spike S1) (Acro, catalogue number RAS-T045), Mouse Anti-SARS-CoV-2 (B.1.351) Antibody IgG Titer Serologic Assay Kit (Spike S1) (Acro, catalogue number RAS-T084), Mouse Anti-SARS-CoV-2 (B.1.617.2) Antibody IgG Titer Serologic Assay Kit (Spike S1) (Acro, catalogue number RAS-T086). The antibody serum samples that yielded optical density values at least 2.1-fold higher than that of the negative control were considered positive. The endpoint titre was defined as the highest serum dilution that yielded a positive optical density value. The geometric mean titre was calculated as the geometric mean of the endpoint titres of the positive serum samples in each group.
Western blotting
Proteins were separated by 12% SDS–PAGE and transferred to polyvinylidene difluoride membranes. The membranes were blocked with 5% bovine serum albumin–Tris-buffered saline with Tween-20 (Sigma-Aldrich) and incubated with an anti-SARS-CoV-2 S1 antibody (MHC0102, Yunnan Lepeng Technology), anti-ACE2 antibody (Abcam, catalogue number ab15348), and anti-DC-SIGN antibody (Santa Cruz Biotechnology, catalogue number sc-74589) for 2 h; washed three times and incubated with HRP-conjugated goat anti-mouse IgG (H + L) (Sigma) for 1 h. Finally, the polyvinylidene difluoride membranes were washed three times and covered with ECL ultrasensitive chemiluminescence reagent (NCM Biotech, catalogue number P10100) and placed in a Bio-Rad gel imager for exposure and colour development.
Silver staining with SDS‒PAGE
The prepared SDS–PAGE gel was silver-stained with a kit (Fast Silver Stain Kit, catalogue number P0017S, Beyotime). Silver staining of the SDS‒PAGE gels was performed in ten steps: fixation, washing with 30% ethanol, washing with water, sensitization, washing with water, silver staining, washing with water, colour development, termination and washing with water.
Flow cytometry analysis
Lymph nodes and spleens were collected on days 3 and 7 after primary and booster immunization with the vaccine, and isolated lymphocyte. The flow-labelled antibodies used to detect the surface markers of DC activation were PE/Cy5-CD45 (catalogue number MA5-38732, Thermo Fisher), PE/Cy7-CD11c (catalogue number A15849, Thermo Fisher), FITC-CD80 (catalogue number A14722, Thermo Fisher), APC-CD83 (catalogue number ab234119, Abcam), and PE-CD86 (catalogue number 12-0862-82, Thermo Fisher). The flow-labelled antibodies used to detect specific T-cell surface markers were BV421-CD44 (catalogue number 103019, BioLegend), APC-CD25 (catalogue number 102011, BioLegend), PE/Cy5-CD3 (catalogue number 100205, BioLegend), FITC-CD4 (catalogue number 100405, BioLegend), APC/Cy7-CD8 (catalogue number 100713, BioLegend) and PE-Tetramer (Helixgen COVID-19 MHC-I Tetramer). The flow-labelled antibodies used to detect activated B-cell surface markers were FITC-CD19 (catalogue number 115505, BioLegend), PerCp/Cy5.5-GL7 (catalogue number 144609, BioLegend) and PE-S1 (Expedeon, catalogue number 336-005). The cells were stained for 30 min at 4 °C and washed twice prior to flow cytometric analysis (LSR Fortessa, BD).
Luminex assays
The Bio-Plex Mouse 23-Plex Panel assay (catalogue number M60009RDPD) was performed according to the manufacturer’s instructions. Briefly, a standard curve ranging from 1.6 to 10,000 pg ml −1 was generated by serial dilution of the reconstituted standard. The filter plates were blocked by pipetting 200 µl of assay buffer into each well. After 10 min, the assay buffer was discarded by vacuum aspiration, and 25 µl of assay diluent was added to the wells designated for the samples, RPMI 1640 with GlutaMAX (Gibco) was added to the wells for the standards. Then, standard or sample was added to the appropriate wells, and 25 µl of antibody-coated fluorescent beads was added. Biotinylated secondary and streptavidin–phycoerythrin-labelled antibodies were subsequently added to the plate through alternating incubation and washing steps. Then, 100 µl of sheath fluid was added to the wells, and read immediately with the Bio-Plex array reader at high and low RP1 targets using a five-parameter logistic regression curve.
Statistical analysis and reproducibility
For western blot and electron microscopy, immunofluorescence assays were repeated at least twice; for ELISA, quantitative analysis was repeated at least three times. All the data are expressed as mean values with s.e.m. Significant differences between groups were analysed by GraphPad Prism. Statistical significance was set to P < 0.05.
Reporting summary
Further information on research design is available in the Nature Portfolio Reporting Summary linked to this article.
Data availability
The main data that support the findings of this study are available within the paper, via figshare at https://doi.org/10.6084/m9.figshare.25531513 (ref. 29 ) and the Supplementary Information . The transcriptomic data were uploaded to GSA (accession number CRA010542 ). Other raw and relevant data from the study are available for research purposes from the corresponding authors upon reasonable request.
Kirtipal, N., Bharadwaj, S. & Kang, S. G. From SARS to SARS-CoV-2, insights on structure, pathogenicity and immunity aspects of pandemic human coronaviruses. Infect. Genet Evol. 85 , 104502 (2020).
Article CAS PubMed PubMed Central Google Scholar
Röltgen, K. et al. Immune imprinting, breadth of variant recognition, and germinal center response in human SARS-CoV-2 infection and vaccination. Cell 185 , 1025–1040.e1014 (2022).
Article PubMed PubMed Central Google Scholar
Vitiello, A., Ferrara, F., Troiano, V. & La Porta, R. COVID-19 vaccines and decreased transmission of SARS-CoV-2. Inflammopharmacology 29 , 1357–1360 (2021).
Lipsitch, M., Krammer, F., Regev-Yochay, G., Lustig, Y. & Balicer, R. D. SARS-CoV-2 breakthrough infections in vaccinated individuals: measurement, causes and impact. Nat. Rev. Immunol. 22 , 57–65 (2022).
Article CAS PubMed Google Scholar
Morens, D. M., Taubenberger, J. K. & Fauci, A. S. Rethinking next-generation vaccines for coronaviruses, influenzaviruses, and other respiratory viruses. Cell Host Microbe 31 , 146–157 (2023).
Verbeke, R., Hogan, M. J., Loré, K. & Pardi, N. Innate immune mechanisms of mRNA vaccines. Immunity 55 , 1993–2005 (2022).
Zheng, B. et al. Inhalable nanovaccine with biomimetic coronavirus structure to trigger mucosal immunity of respiratory tract against COVID-19. Chem. Eng. J. 418 , 129392 (2021).
Jackson, C. B., Farzan, M., Chen, B. & Choe, H. Mechanisms of SARS-CoV-2 entry into cells. Nat. Rev. Mol. Cell Biol. 23 , 3–20 (2022).
Chang, T. et al. Depletion and dysfunction of dendritic cells: understanding SARS-CoV-2 infection. Front Immunol. 13 , 843342 (2022).
Labzin, L. I. et al. Macrophage ACE2 is necessary for SARS-CoV-2 replication and subsequent cytokine responses that restrict continued virion release. Sci. Signal 16 , eabq1366 (2023).
Eygeris, Y., Gupta, M., Kim, J. & Sahay, G. Chemistry of lipid nanoparticles for RNA delivery. Acc. Chem. Res. 55 , 2–12 (2022).
Haley, R. M. et al. Lipid nanoparticle delivery of small proteins for potent in vivo RAS inhibition. ACS Appl. Mater. Interfaces 15 , 21877–21892 (2023).
Cai, G. et al. SARS-CoV-2 impairs dendritic cells and regulates DC-SIGN gene expression in tissues. Int. J. Mol. Sci. 22 , 9228 (2021).
Brufsky, A. & Lotze, M. T. DC/L-SIGNs of hope in the COVID-19 pandemic. J. Med Virol. 92 , 1396–1398 (2020).
Soilleux, E. J. et al. Constitutive and induced expression of DC-SIGN on dendritic cell and macrophage subpopulations in situ and in vitro. J. Leukoc. Biol. 71 , 445–457 (2002).
Hato, T. & Dagher, P. C. How the innate immune system senses trouble and causes trouble. Clin. J. Am. Soc. Nephrol. 10 , 1459–1469 (2015).
Ishii, K. J., Koyama, S., Nakagawa, A., Coban, C. & Akira, S. Host innate immune receptors and beyond: making sense of microbial infections. Cell Host Microbe 3 , 352–363 (2008).
Radmand, A. et al. The transcriptional response to lung-targeting lipid nanoparticles in vivo. Nano Lett. 23 , 993–1002 (2023).
Ndeupen, S. et al. The mRNA-LNP platform’s lipid nanoparticle component used in preclinical vaccine studies is highly inflammatory. iScience 24 , 103479 (2021).
Park, Y. et al. Poly(aspartic acid)-based polymeric nanoparticle for local and systemic mRNA delivery. Mol. Pharm. 19 , 4696–4704 (2022).
Marsman, C. et al. Plasmacytoid dendritic cell heterogeneity is defined by CXCL10 expression following TLR7 stimulation. Immunol. Cell Biol. 96 , 1083–1094 (2018).
Naumova, E. N. Public health response to COVID-19: the forecaster’s dilemma. J. Public Health Policy 41 , 395–398 (2020).
Huyser, K. R., Horse, A. J. Y., Kuhlemeier, A. A. & Huyser, M. R. COVID-19 pandemic and indigenous representation in public health data. Am. J. Public Health 111 , S208–s214 (2021).
Li, M. et al. COVID-19 vaccine development: milestones, lessons and prospects. Signal Transduct. Target Ther. 7 , 146 (2022).
Gómez-Aguado, I. et al. mRNA delivery technologies: toward clinical translation. Int. Rev. Cell Mol. Biol. 372 , 207–293 (2022).
Article PubMed Google Scholar
Takeda, K. & Akira, S. Toll-like receptors. Curr. Protoc. Immunol. 109 , 14.12.11–14.12.10 (2015).
Article Google Scholar
Kaisho, T. Molecular mechanisms for plasmacytoid dendritic cell function and development. Vaccine 28 , 8046–8047 (2010).
Mendoza, E. J., Manguiat, K., Wood, H. & Drebot, M. Two detailed plaque assay protocols for the quantification of infectious SARS-CoV-2. Curr. Protoc. Microbiol. 57 , ecpmc105 (2020).
Zhang, J. et al. Virus-like structures for combination antigen protein mRNA vaccination. figshare , https://doi.org/10.6084/m9.figshare.25531513 (2024).
Download references
Acknowledgements
We thank the Institutional Center for Shared Technologies and Facilities of Kunming Institute of Zoology (KIZ), Chinese Academy of Sciences (CAS), for providing us with the transmission electron microscope. We are grateful to Y. Guo and X. Wu for their help with preparing the electron microscopy samples and taking/analysing electron microscopy images.
Author information
These authors contributed equally: Jingjing Zhang, Yanmei Li.
Authors and Affiliations
Weirui Biotechnology (Kunming) Co., Ltd, Ciba Biotechnology Innovation Center, Kunming, China
Jingjing Zhang, Yanmei Li, Fengyuan Zeng, Changyong Mu, Change Liu, Lichun Wang, Xiaowu Peng, Liping He, Yanrui Su, Hongbing Li, An Wang, Lin Feng, Dongxiu Gao, Zhixiao Zhang, Gang Xu, Yixuan Wang, Rong Yue, Junbo Si, Lichun Zheng, Xiong Zhang, Fuyun He, Hongkun Yi, Zhongshu Tang, Gaocan Li, Kaili Ma & Qihan Li
Shandong WeigaoLitong Biological Products Co., Ltd, Weihai, China
Jingjing Zhang & Kaili Ma
You can also search for this author in PubMed Google Scholar
Contributions
Q.L. and K.M. conceived and designed the study. J.Z., Y.L., C.M., L.W., C.L. and X.P. performed the research. R.Y., F.Z., L.H., Y.S., H.L., A.W., L.F. and D.G. contributed reagents. Z.Z., G.X., Y.W., F.H. and J.S. contributed materials. J.Z., L.Z., X.Z., H.Y., Z.T., G.L., Q.L. and K.M. analysed the data. Q.L. and J.Z. wrote the first draft. All authors contributed to the article and approved the submitted version.
Corresponding authors
Correspondence to Kaili Ma or Qihan Li .
Ethics declarations
Competing interests.
The authors declare no competing interests.
Peer review
Peer review information.
Nature Nanotechnology thanks Michael Mitchell, Adam Sander and the other, anonymous, reviewer(s) for their contribution to the peer review of this work.
Additional information
Publisher’s note Springer Nature remains neutral with regard to jurisdictional claims in published maps and institutional affiliations.
Extended data
Extended data fig. 1 the speculated mechanism of vlss vaccines interacting with host immune system and eliciting immune response in mice..
➀ Injected VLSs vaccines are endocytosed by the epithelial cells. After escaping from the endosome and entering the cytosol, mRNA is translated into protein by the ribosome. The translated antigenic protein can be secreted into the extracellular space. DCs and macrophages can be recruited by the local inflammation induced by VLSs. Both the secreted antigens and the antigens from the VLSs vaccine surface can be taken up by the recruited DCs and macrophages, followed by their activation and presentation to Tfh/Th1 and B cells. ➁ The injected VLSs vaccines are endocytosed by DCs and macrophages by the mediation of S1 protein binding specifically with DC-sign or ACE2 receptor. The delivered mRNA is translated into protein as an endogenous antigen that can activate DCs and macrophages. These activated immune cells enable antigen presentation to Tfh/Th1 and B cells for specific immunity activation and specific proliferation of B cells for antibody responses, while raising the specific CTL response. ➂ S1 protein antigen in the surface of VLSs vaccines, as an exogenous antigen, is recognized and endocytosed by DCs and macrophages, followed by their activation and antigen presentation to Tfh/Th1 and B cells. All antigenic signals from these pathways can activate and improve specific immune response.
Supplementary information
Supplementary information.
Supplementary Figs. 1–10.
Reporting Summary
Rights and permissions.
Open Access This article is licensed under a Creative Commons Attribution 4.0 International License, which permits use, sharing, adaptation, distribution and reproduction in any medium or format, as long as you give appropriate credit to the original author(s) and the source, provide a link to the Creative Commons licence, and indicate if changes were made. The images or other third party material in this article are included in the article’s Creative Commons licence, unless indicated otherwise in a credit line to the material. If material is not included in the article’s Creative Commons licence and your intended use is not permitted by statutory regulation or exceeds the permitted use, you will need to obtain permission directly from the copyright holder. To view a copy of this licence, visit http://creativecommons.org/licenses/by/4.0/ .
Reprints and permissions
About this article
Cite this article.
Zhang, J., Li, Y., Zeng, F. et al. Virus-like structures for combination antigen protein mRNA vaccination. Nat. Nanotechnol. (2024). https://doi.org/10.1038/s41565-024-01679-1
Download citation
Received : 02 July 2023
Accepted : 15 April 2024
Published : 27 May 2024
DOI : https://doi.org/10.1038/s41565-024-01679-1
Share this article
Anyone you share the following link with will be able to read this content:
Sorry, a shareable link is not currently available for this article.
Provided by the Springer Nature SharedIt content-sharing initiative
Quick links
- Explore articles by subject
- Guide to authors
- Editorial policies
Sign up for the Nature Briefing: Translational Research newsletter — top stories in biotechnology, drug discovery and pharma.


IMAGES
VIDEO
COMMENTS
Nature Reviews Immunology (2024) Antigen processing and presentation are the cornerstones of adaptive immunity. B cells cannot generate high-affinity antibodies without T cell help. CD4+ T cells ...
MHC complexes. Antigen processing and presentation enable the adaptive immune system to survey the host cell proteome and detect pathogens and mutations 36,37.MHC I and MHC II are the two ...
Abstract. Antigen processing and presentation are the cornerstones of adaptive immunity. B cells cannot generate high-affinity antibodies without T cell help. CD4 + T cells, which provide such help, use antigen-specific receptors that recognize major histocompatibility complex (MHC) molecules in complex with peptide cargo.
Nature Reviews Immunology - The pathways of antigen processing and presentation are well known, but how do antigens gain access to MHC molecules in dendritic cells and what is the role of ...
Abstract | Antigen processing and presentation are the cornerstones of adaptive immunity. B cells cannot generate high- affinity antibodies without T cell help. CD4 T cells, which provide. such ...
Nature reviews. Immunology, 13 Apr 2022, 22(12): 751-764 ... Antigen processing and presentation are the cornerstones of adaptive immunity. B cells cannot generate high-affinity antibodies without T cell help. CD4 + T cells, which provide such help, use antigen-specific receptors that recognize major histocompatibility complex ...
The principal components of both MHC class I and class II antigen processing and presentation pathways are well known. In dendritic cells, these pathways are tightly regulated by Toll-like-receptor signalling and include features, such as cross-presentation, that are not seen in other cell types. However, the exact mechanisms involved in the ...
1. The Immune System and Cancer. The interplay between the immune system and cancer termed "cancer immunoediting" is a dynamic and continuously evolving process in which immune responses can eradicate tumor cells but also promote tumor progression through selective pressures [1,2].The temporal evolution of the immune system-cancer interaction is usually considered to consist of at least ...
In this Review, we describe the molecular mechanisms of antigen processing and presentation in tumours and their clinical consequences. We examine how various aspects of the antigen-presentation machinery (APM) shape tumour immunity. In particular, we discuss genomic variants in HLA alleles and other APM components, highlighting their influence ...
This 'guide to' article provides an overview of the antigen processing and presentation pathways, which lead to the loading of antigenic peptides on major histocompatibility complex (MHC) molecules for detection by T cells. Antigen processing and presentation are the cornerstones of adaptive immunity. B cells cannot generate high-affinity antibodies without T cell help. CD4+ T cells, which ...
John Trowsdale and Adrian Kelly (Kelly & Trowsdale 2019) review a history of the genetic approaches, both traditional and modern, that have been highly successful in the quest to understand antigen processing and presentation. Peter Cresswell's review (Cresswell 2019) is a personal retrospective focusing on the biochemistry of antigen ...
Read the latest Research articles in Antigen processing and presentation from Nature Reviews Genetics. ... Nature Reviews Genetics (Nat Rev Genet) ISSN 1471-0064 (online ...
T cell recognition of antigen-presenting cells depends on their expression of a spectrum of peptides bound to major histocompatibility complex class I (MHC-I) and class II (MHC-II) molecules. Conversion of antigens from pathogens or transformed cells into MHC-I- and MHC-II-bound peptides is critical for mounting protective T cell responses, and similar processing of self proteins is necessary ...
In this review, we discuss the ... Among the "non-classical" routes for antigen processing and presentation, antigen cross-presentation has received the most attention during the last 20 years. ... CD8 + T cell responses can be generated either by direct- or cross-presentation, which depends on the nature of the microbe. For instance, anti ...
Processing of the antigen, to peptides or other moieties, requires other sets of molecules. For classical class I, this includes TAP peptide transporters, proteasome components and Tapasin, genes which are encoded within the MHC. Similarly, HLA-DO and -DM, which influence presentation by HLA class II molecules, are encoded in the MHC region.
Two papers present recent advances in the field of antigen presentation to TCR invariant T cells. Vartabedian et al. 9 draw a broad picture of the biology of antigen presentation of lipids and glycolipids by CD1d molecules to NKT cells. They describe the intracellular traffic of CD1d, and review the structure and processing of the various ...
Immunology 2022 December. Antigen processing and presentation are the cornerstones of adaptive immunity. B cells cannot generate high-affinity antibodies without T cell help. CD4+ T cells, which provide such help, use antigen-specific receptors that recognize major histocompatibility complex (MHC) molecules in complex with peptide cargo.
Here, we review the tumour-derived factors modulating DC function, and we summarize evidence of immune evasion by means of quantitative modulation or qualitative alteration of the antigen repertoire presented on tumours. ... HLA-I surface levels, alterations in the antigen processing and presentation machinery in tumour cells. Lastly, as ...
The mechanisms of antigen processing and presentation play a crucial role in the recognition and targeting of cancer cells by the immune system. Cancer cells can evade the immune system by downregulating or losing the expression of the proteins recognized by the immune cells as antigens, creating an immunosuppressive microenvironment, and altering their ability to process and present antigens ...
In this Review, the authors describe the mechanisms of synergy between chemotherapy and immune checkpoint inhibitors, summarize the available clinical data on these effects and highlight the most ...
Antigen presentation is an essential immunological mechanism for T-cell immune response stimulation. Only when antigens are exposed on cell surfaces can the T cells recognize them, the important of which are dendritic cells, B cells, and macrophages. ... Antigen Processing Cells. In: Dictionary of Toxicology. Springer, Singapore. https://doi ...
Immune response to disease requires coordinated expression of an army of molecules. The highly polymorphic MHC class I and class II molecules are key to control of specificity of antigen presentation. Processing of the antigen, to peptides or other moieties, requires other sets of molecules. For classical class I, this includes TAP peptide transporters, proteasome components and Tapasin, genes ...
This type of presentation elicits a weak immune response. To increase the strength of the immune response, some DDS for mRNA vaccines have enabled antigen cross-presentation by facilitating the escape of antigens from lysosomes or endosomes . Cross-presentation can be enabled in three main ways: (1) Proton sponge effect.
The mechanisms of antigen uptake, the nature of the antigen processing compartments and the lifetime of cell surface peptide-MHC class II complexes can vary depending on the type of APC.
Nowadays, immunotherapy is one of the most promising anti-tumor therapeutic strategy. Specifically, immune-related targets can be used to predict the efficacy and side effects of immunotherapy and monitor the tumor immune response. In the past few decades, increasing numbers of novel immune biomarkers have been found to participate in certain links of the tumor immunity to contribute to the ...
The field of antigen processing and presentation has continued to advance since the publication of a focus issue on the topic in Nature Immunology in July 2004. Progress has been made on many ...
Therapeutic modified mRNAs are being developed for a broad range of human diseases. However, the impact of potential miscoding of modified mRNAs on self-tolerance remains unknown. Additionally, more studies are needed to explore the effects of nucleoside alkylation on translation. While all six tested modifications are tolerated as substrates by T7 RNA polymerase and inhibited mRNA ...
This paper presents a virus-like lipid nanoparticle decorated with spike proteins capable of carrying antigens, including mRNA and proteins, for vaccination against SARS-CoV-2 variants.