18.4 Electric Potential
Section learning objectives.
By the end of this section, you will be able to do the following:
- Explain the similarities and differences between electric potential energy and gravitational potential energy
- Calculate the electric potential difference between two point charges and in a uniform electric field

Teacher Support
The learning objectives in this section will help your students master the following standards:
- (C) describe and calculate how the magnitude of the electrical force between two objects depends on their charges and the distance between them; and
- (D) identify examples of electric and magnetic forces in everyday life.
Section Key Terms
This section again uses the analogy between gravity and electric force to present electric potential energy and electric potential.
[BL] Ask students to define how potential is used in everyday life. Draw the analogy between this and potential energy.
[OL] [AL] Review the concept of potential energy as being the potential an object has to do work by virtue of its position with respect to another object.
[OL] Remind students that gravitational potential energy is only meaningful when we deal with differences in energy. In other words, a reference position is chosen, and all other potential energies are compared with the potential energy at the reference position.
[AL] Point out that sometimes the reference position is at infinity, in which case this may not be explicitly stated.
As you learned in studying gravity, a mass in a gravitational field has potential energy , which means it has the potential to accelerate and thereby increase its kinetic energy . This kinetic energy can be used to do work. For example, imagine you want to use a stone to pound a nail into a piece of wood. You first lift the stone high above the nail, which increases the potential energy of the stone-Earth system—because Earth is so large, it does not move, so we usually shorten this by saying simply that the potential energy of the stone increases. When you drop the stone, gravity converts the potential energy into kinetic energy. When the stone hits the nail, it does work by pounding the nail into the wood. The gravitational potential energy is the work that a mass can potentially do by virtue of its position in a gravitational field. Potential energy is a very useful concept, because it can be used with conservation of energy to calculate the motion of masses in a gravitational field.
Electric potential energy works much the same way, but it is based on the electric field instead of the gravitational field. By virtue of its position in an electric field, a charge has an electric potential energy. If the charge is free to move, the force due to the electric field causes it to accelerate, so its potential energy is converted to kinetic energy, just like a mass that falls in a gravitational field. This kinetic energy can be used to do work. The electric potential energy is the work that a charge can do by virtue of its position in an electric field.
The analogy between gravitational potential energy and electric potential energy is depicted in Figure 18.21 . On the left, the ball-Earth system gains gravitational potential energy when the ball is higher in Earth's gravitational field. On the right, the two-charge system gains electric potential energy when the positive charge is farther from the negative charge.
Let’s use the symbol U G U G to denote gravitational potential energy. When a mass falls in a gravitational field, its gravitational potential energy decreases. Conservation of energy tells us that the work done by the gravitational field to make the mass accelerate must equal the loss of potential energy of the mass. If we use the symbol W done by gravity W done by gravity to denote this work, then
where the minus sign reflects the fact that the potential energy of the ball decreases.
The work done by gravity on the mass is
where F is the force due to gravity, and y i y i and y f y f are the initial and final positions of the ball, respectively. The negative sign is because gravity points down, which we consider to be the negative direction. For the constant gravitational field near Earth’s surface, F = m g F = m g . The change in gravitational potential energy of the mass is
Note that y f − y i y f − y i is just the negative of the height h from which the mass falls, so we usually just write Δ U G = − m g h Δ U G = − m g h .
We now apply the same reasoning to a charge in an electric field to find the electric potential energy. The change Δ U E Δ U E in electric potential energy is the work done by the electric field to move a charge q from an initial position x i x i to a final position x f x f ( − Δ U E = W done by E-field − Δ U E = W done by E-field ). The definition of work does not change, except that now the work is done by the electric field: W done by E-field = F ( x f − x i ) W done by E-field = F ( x f − x i ) . For a charge that falls through a constant electric field E , the force applied to the charge by the electric field is F = q E F = q E . The change in electric potential energy of the charge is thus
This equation gives the change in electric potential energy of a charge q when it moves from position x i x i to position x f x f in a constant electric field E .
Figure 18.22 shows how this analogy would work if we were close to Earth’s surface, where gravity is constant. The top image shows a charge accelerating due to a constant electric field. Likewise, the round mass in the bottom image accelerates due to a constant gravitation field. In both cases, the potential energy of the particle decreases, and its kinetic energy increases.
Point out the fringing fields at the ends of the plates. Ask what path the charge would take if it were released in a fringing field (note that the charge does not follow the field lines due to its inertia).
Ask students to draw the gravitational field lines in the bottom picture. Discuss why the mass does not follow the gravitational field lines.
Watch Physics
Analogy between gravity and electricity.
This video discusses the analogy between gravitational potential energy and electric potential energy. It reviews the concepts of work and potential energy and shows the connection between a mass in a uniform gravitation field, such as on Earth’s surface, and an electric charge in a uniform electric field.
If the electric field is not constant, then the equation Δ U E = − q E ( x f − x i ) Δ U E = − q E ( x f − x i ) is not valid, and deriving the electric potential energy becomes more involved. For example, consider the electric potential energy of an assembly of two point charges q 1 q 1 and q 2 q 2 of the same sign that are initially very far apart. We start by placing charge q 1 q 1 at the origin of our coordinate system. This takes no electrical energy, because there is no electric field at the origin (because charge q 2 q 2 is very far away). We then bring charge q 2 q 2 in from very far away to a distance r from the center of charge q 1 q 1 . This requires some effort, because the electric field of charge q 1 q 1 applies a repulsive force on charge q 2 q 2 . The energy it takes to assemble these two charges can be recuperated if we let them fly apart again. Thus, the charges have potential energy when they are a distance r apart. It turns out that the electric potential energy of a pair of point charges q 1 q 1 and q 2 q 2 a distance r apart is
To recap, if charges q 1 q 1 and q 2 q 2 are free to move, they can accumulate kinetic energy by flying apart, and this kinetic energy can be used to do work. The maximum amount of work the two charges can do (if they fly infinitely far from each other) is given by the equation above.
Notice that if the two charges have opposite signs, then the potential energy is negative. This means that the charges have more potential to do work when they are far apart than when they are at a distance r apart. This makes sense: Opposite charges attract, so the charges can gain more kinetic energy if they attract each other from far away than if they start at only a short distance apart. Thus, they have more potential to do work when they are far apart. Figure 18.23 summarizes how the electric potential energy depends on charge and separation.
Have students imagine that they have to push together or pull apart balls that carry these charges. Discuss which would require more work: pushing them together from far apart or from close together. Compare this with the analogous situations for the different charge combinations and for pushing together versus pulling apart.
Electric Potential
Recall that to find the force applied by a fixed charge Q on any arbitrary test charge q , it was convenient to define the electric field, which is the force per unit charge applied by Q on any test charge that we place in its electric field. The same Strategy is used here with electric potential energy: We now define the electric potential V , which is the electric potential energy per unit charge.
Misconception Alert
Emphasize the difference between electric potential energy and electric potential. Although the latter seems to be shorthand for the former, the two terms have different meanings.
Normally, the electric potential is simply called the potential or voltage . The units for the potential are J/C, which are given the name volt (V) after the Italian physicist Alessandro Volta (1745–1827). From the equation U E = k q 1 q 2 / r U E = k q 1 q 2 / r , the electric potential a distance r from a point charge q 1 q 1 is
This equation gives the energy required per unit charge to bring a charge q 2 q 2 from infinity to a distance r from a point charge q 1 . q 1 . Mathematically, this is written as
Note that this equation actually represents a difference in electric potential. However, because the second term is zero, it is normally not written, and we speak of the electric potential instead of the electric potential difference , or we just say the potential difference, or voltage). Below, when we consider the electric potential energy per unit charge between two points not infinitely far apart, we speak of electric potential difference explicitly. Just remember that electric potential and electric potential difference are really the same thing; the former is used just when the electric potential energy is zero in either the initial or final charge configuration.
Coming back now to the electric potential a distance r from a point charge q 1 q 1 , note that q 1 q 1 can be any arbitrary point charge, so we can drop the subscripts and simply write
Now consider the electric potential near a group of charges q 1 , q 2 , and q 3 , as drawn in Figure 18.24 . The electric potential is derived by considering the electric field. Electric fields follow the principle of superposition and can be simply added together, so the electric potential from different charges also add together. Thus, the electric potential of a point near a group of charges is
where r 1 , r 2 , r 3 , …, r 1 , r 2 , r 3 , …, are the distances from the center of charges q 1 , q 2 , q 3 , … q 1 , q 2 , q 3 , … to the point of interest, as shown in Figure 18.24 .
Now let’s consider the electric potential in a uniform electric field. From the equation Δ U E = − q E ( x f − x i ) Δ U E = − q E ( x f − x i ) , we see that the potential difference in going from x i x i to x f x f in a uniform electric field E is
Tips For Success
Notice from the equation Δ V = − E ( x f − x i ) Δ V = − E ( x f − x i ) that the electric field can be written as
which means that the electric field has units of V/m. Thus, if you know the potential difference between two points, calculating the electric field is very simple—you simply divide the potential difference by the distance!
Notice that a positive charge in a region with high potential will experience a force pushing it toward regions of lower potential. In this sense, potential is like pressure for fluids. Imagine a pipe containing fluid, with the fluid at one end of the pipe under high pressure and the fluid at the other end of the pipe under low pressure. If nothing prevents the fluid from flowing, it will flow from the high-pressure end to the low-pressure end. Likewise, a positive charge that is free to move will move from a region with high potential to a region with lower potential.
This video starts from electric potential energy and explains how this is related to electric potential (or voltage). The lecturer calculates the electric potential created by a uniform electric field.
Links To Physics
Electric animals.
Many animals generate and/or detect electric fields. This is useful for activities such as hunting, defense, navigation, communication, and mating. Because salt water is a relatively good conductor, electric fish have evolved in all the world’s oceans. These fish have intrigued humans since the earliest times. In the nineteenth century, parties were even organized where the main attraction was getting a jolt from an electric fish! Scientists also studied electric fish to learn about electricity. Alessandro Volta based his research that led to batteries in 1799 on electric fish. He even referred to batteries as artificial electric organs, because he saw them as imitations of the electric organs of electric fish.
Animals that generate electricity are called electrogenic and those that detect electric fields are called electroreceptive . Most fish that are electrogenic are also electroreceptive. One of the most well-known electric fish is the electric eel (see Figure 18.25 ), which is both electrogenic and electroreceptive. These fish have three pairs of organs that produce the electric charge: the main organ, Hunter’s organ, and Sach’s organ. Together, these organs account for more than 80percent of the fish’s body.
Electric eels can produce electric discharges of much greater voltage than what you would get from a standard wall socket. These discharges can stun or even kill their prey. They also use low-intensity discharges to navigate. The electric fields they generate reflect off nearby obstacles or animals and are then detected by electroreceptors in the eel’s skin. The three organs that produce electricity contain electrolytes, which are substances that ionize when dissolved in water (or other liquids). An ionized atom or molecule is one that has lost or gained at least one electron, so it carries a net charge. Thus, a liquid solution containing an electrolyte conducts electricity, because the ions in the solution can move if an electric field is applied.
To produce large discharges, the main organ is used. It contains approximately 6,000 rows of electroplaques connected in a long chain. Connected this way, the voltage between electroplaques adds up, creating a large final voltage. Each electroplaque consists of a column of cells controlled by an excitor nerve. When triggered by the excitor nerve, the electroplaques allow ionized sodium to flow through them, creating a potential difference between electroplaques. These potentials add up, and a large current can flow through the electrolyte.
This geometry is reflected in batteries, which also use stacks of plates to produce larger potential differences.
Grasp Check
If an electric eel produces 1,000 V, which voltage is produced by each electroplaque in the main organ?
Worked Example
Dentists use X-rays to image their patients’ teeth and bones. The X-ray tubes that generate X-rays contain an electron source separated by about 10 cm from a metallic target. The electrons are accelerated from the source to the target by a uniform electric field with a magnitude of about 100 kN/C, as drawn in Figure 18.26 . When the electrons hit the target, X-rays are produced. (a) What is the potential difference between the electron source and the metallic target? (b) What is the kinetic energy of the electrons when they reach the target, assuming that the electrons start at rest?
Strategy FOR (A)
Use the equation Δ V = − E ( x f − x i ) Δ V = − E ( x f − x i ) to find the potential difference given a constant electric field. Define the source position as x i = 0 x i = 0 and the target position as x f = 10 cm x f = 10 cm . To accelerate the electrons in the positive x direction, the electric field must point in the negative x direction. This way, the force F = q E F = q E on the electrons will point in the positive x direction, because both q and E are negative. Thus, E = − 100 × 10 3 N/C E = − 100 × 10 3 N/C .
Using x i = 0 x i = 0 and x f = 10 cm = 0.10 m x f = 10 cm = 0.10 m , the equation Δ V = − E ( x f − x i ) Δ V = − E ( x f − x i ) tells us that the potential difference between the electron source and the target is
The potential difference is positive, so the energy per unit positive charge is higher at the target than at the source. This means that free positive charges would fall from the target to the source. However, electrons are negative charges, so they accelerate from the source toward the target, gaining kinetic energy as they go.
Strategy FOR (B)
Apply conservation of energy to find the final kinetic energy of the electrons. In going from the source to the target, the change in electric potential energy plus the change in kinetic energy of the electrons must be zero, so Δ U E + Δ K = 0 . Δ U E + Δ K = 0 . The change in electric potential energy for moving through a constant electric field is given by the equation
Δ U E = − q E ( x f − x i ) , Δ U E = − q E ( x f − x i ) ,
where the electric field is E = − 100 × 10 3 N/C E = − 100 × 10 3 N/C . Because the electrons start at rest, their initial kinetic energy is zero. Thus, the change in kinetic energy is simply their final kinetic energy, so Δ K = K f Δ K = K f .
Again x i = 0 x i = 0 and x f = 10 cm = 0.10 m x f = 10 cm = 0.10 m . The charge of an electron is q = − 1.602 × 10 − 19 C q = − 1.602 × 10 − 19 C . Conservation of energy gives
Inserting the known values into the right-hand side of this equation gives
This is a very small energy. However, electrons are very small, so they are easy to accelerate, and this energy is enough to make an electron go extremely fast. You can find their speed by using the definition of kinetic energy, K = 1 2 m v 2 K = 1 2 m v 2 . The result is that the electrons are moving at more than 100 million miles per hour!
Electric Potential Energy of Doorknob and Dust Speck
Consider again the doorknob from the example in the previous section. The doorknob is treated as a spherical conductor with a uniform static charge q 1 = −1.5 nC q 1 = −1.5 nC on its surface. What is the electric potential energy between the doorknob and a speck of dust carrying a charge q 2 = 0.20 nC q 2 = 0.20 nC at 1.0 cm from the front surface of the doorknob? The diameter of the doorknob is 5.0 cm.
As we did in the previous section, we treat the charge as if it were concentrated at the center of the doorknob. Again, as you will be able to validate in later physics classes, we can make this simplification, because the charge is uniformly distributed over the surface of the spherical object. Make a sketch of the situation and define a coordinate system, as shown in the image below. We use + x + x to indicate the outward direction perpendicular to the door, with x = 0 x = 0 at the center of the doorknob. If the diameter of the doorknob is 5.0 cm, its radius is 2.5 cm. Thus, the speck of dust 1.0 cm from the surface of the doorknob is a distance r = 2.5 cm + 1.0 cm = 3.5 cm r = 2.5 cm + 1.0 cm = 3.5 cm from the center of the doorknob. To solve this problem, use the equation U E = k q 1 q 2 / r U E = k q 1 q 2 / r .
The charge on the doorknob is q 1 = −1.5 nC = −1.5 × 10 −9 C, q 1 = −1.5 nC = −1.5 × 10 −9 C, and the charge on the speck of dust is q 2 = 0.20 nC = 2.0 × 10 −10 C q 2 = 0.20 nC = 2.0 × 10 −10 C . The distance r = 3.5 cm = 0.035 m r = 3.5 cm = 0.035 m . Inserting these values into the equation U E = k q 1 q 2 / r U E = k q 1 q 2 / r gives
The energy is negative, which means that the energy will decrease that is, get even more negative as the speck of dust approaches the doorknob. This helps explain why dust accumulates on objects that carry a static charge. However, note that insulators normally collect more static charge than conductors, because any charge that accumulates on insulators cannot move about on the insulator to find a way to escape. They must simply wait to be removed by some passing moist speck of dust or other host.
Practice Problems
What is the electric potential 10 cm from a −10 nC charge?
- 9.0 × 10 2 V
- 9.0 × 10 3 V
- 9.0 × 10 4 V
- 9.0 × 10 5 V
An electron accelerates from 0 to 10 × 10 4 m/s in an electric field. Through what potential difference did the electron travel? The mass of an electron is 9.11 × 10 –31 kg, and its charge is −1.60 × 10 –19 C.
Check Your Understanding
Gravitational potential energy is the potential for two masses to do work by virtue of their positions with respect to each other. What is the analogous definition of electric potential energy?
- Electric potential energy is the potential for two charges to do work by virtue of their positions with respect to the origin point.
- Electric potential energy is the potential for two charges to do work by virtue of their positions with respect to infinity.
- Electric potential energy is the potential for two charges to do work by virtue of their positions with respect to each other.
- Electric potential energy is the potential for single charges to do work by virtue of their positions with respect to their final positions.
A negative charge is 10 m from a positive charge. Where would you have to move the negative charge to increase the potential energy of the system?
- The negative charge should be moved closer to the positive charge.
- The negative charge should be moved farther away from the positive charge.
- The negative charge should be moved to infinity.
- The negative charge should be placed just next to the positive charge.
As an Amazon Associate we earn from qualifying purchases.
This book may not be used in the training of large language models or otherwise be ingested into large language models or generative AI offerings without OpenStax's permission.
Want to cite, share, or modify this book? This book uses the Creative Commons Attribution License and you must attribute Texas Education Agency (TEA). The original material is available at: https://www.texasgateway.org/book/tea-physics . Changes were made to the original material, including updates to art, structure, and other content updates.
Access for free at https://openstax.org/books/physics/pages/1-introduction
- Authors: Paul Peter Urone, Roger Hinrichs
- Publisher/website: OpenStax
- Book title: Physics
- Publication date: Mar 26, 2020
- Location: Houston, Texas
- Book URL: https://openstax.org/books/physics/pages/1-introduction
- Section URL: https://openstax.org/books/physics/pages/18-4-electric-potential
© Jan 19, 2024 Texas Education Agency (TEA). The OpenStax name, OpenStax logo, OpenStax book covers, OpenStax CNX name, and OpenStax CNX logo are not subject to the Creative Commons license and may not be reproduced without the prior and express written consent of Rice University.
Electric Potential
Claimed by Neal Austensen Fall2023
- 1.1 A Mathematical Model
- 1.2 A Computational Model
- 2.2 Middling
- 2.3 Difficult
- 3 Connectedness
- 5.1 Further reading
- 5.2 Externals links
- 6 References
- 7 The Main Idea
- 8.1 Middling
- 8.2 Difficult
- 9 Connectedness
- 11.1 External Links
- 12 References
The Main Idea
Electric Potential Energy , like all forms of potential energy, is the potential for work to be done, in this case by the electric force. The Electric Potential (frequently referred to as voltage, from its SI unit, the Volt) is the Electric Potential Energy associated with the test charge (1 Coulomb), such that it depends only on the source, just as the electric field is related to the electric force, but depends only on the source. One may similarly remember the parallel concept of the gravitational potential, which was gravitational potential energy divided by mass.
A Mathematical Model
Electric Potential Energy is defined with respect to the Electric Force & Electric Field :
The differential work [math]\displaystyle{ (dW) }[/math] associated with an external force [math]\displaystyle{ (\mathbf{F}_{ext}) }[/math] moving a charge [math]\displaystyle{ (q) }[/math] from point b to point a [math]\displaystyle{ (d\mathbf{L}) }[/math] through an electric field [math]\displaystyle{ (\mathbf{E}) }[/math] is:
The external force must be equal and opposite to the force associated with the source charge's electric field:
Integrating from point b to point a along the path gives:
Finally, since the work was defined externally, the calculated work is equal to the Electric Potential Energy :
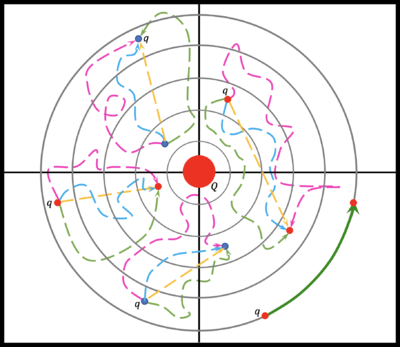
From this, the Electric Potential is defined as the Electric Potential Energy per test charge in the source charge's electric field:
- The electric field is a conservative vector field :
- A special case shown in dark green near the bottom right of the figure also exists:
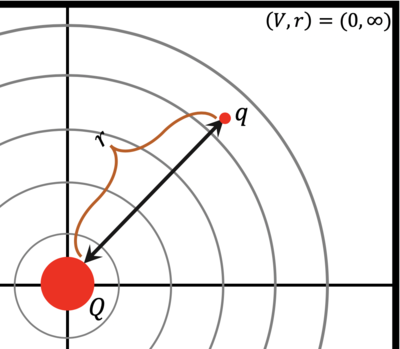
- The Electric Potential from a source charge [math]\displaystyle{ (Q) }[/math] can be written in a simpler expression:
- The initial position is often taken to be infinity (the Electric Potential is defined to be zero at infinity) creating a simpler and more familiar looking equation:
If a charge [math]\displaystyle{ (q) }[/math] is being moved in a source charge's [math]\displaystyle{ (Q) }[/math] electric field [math]\displaystyle{ (\mathbf{E}) }[/math] the Electric Potential Energy is:
The electric field is known to be described radially as: ( Electric Field )
Allow the path be along the radial direction:
Plugging these in gives:
Therefore, the Electric Potential is:
- Critical Formulas
A Computational Model
Click on the link to see Electric Potential through VPython! Make sure to press "Run" to see the principle in action!
Click the link below and press run to see a visual model of electric potential in space with equal and opposite charges (Press "Run")
Watch this video for a more visual approach!
A parallel plate capacitor is shown below:
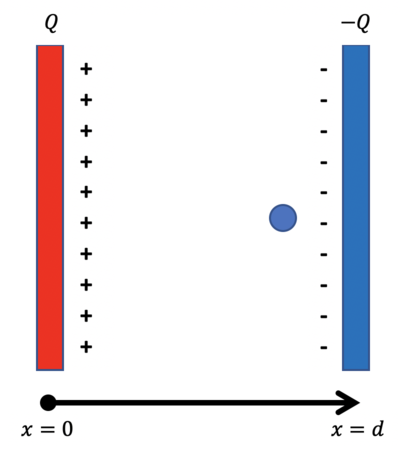
Each plate has area [math]\displaystyle{ A }[/math] with positive charge [math]\displaystyle{ Q }[/math] on the left plate and negative charge [math]\displaystyle{ -Q }[/math] on the right plate. The distance between the plates is [math]\displaystyle{ d }[/math] .
- Question 1 :
Electric fields always point from positive to negative charge. Therefore, the electric field between the plates will point in the positive [math]\displaystyle{ x }[/math] -direction, straight from the left plate to the right plate since the distribution of positive and negative charge is flat and symmetric. The electric field will bend near the edges (called fringing fields). These are usually neglected if [math]\displaystyle{ d }[/math] is much smaller than the length of the plates.
- Question 2 :
We start with noting that the charge ( [math]\displaystyle{ q_n=-\alpha q, \alpha \gt 0 }[/math] ) in question is a negative charge. This effectively means all analysis will be reversed i.e. if the electric potential energy was increasing for a positive charge, it would be decreasing for a negative charge. With that said, we can start by noticing that the charge is moving against the electric field between the plates. Since this a negative charge, this is the way that negative charge wants to move, meaning it is losing electric potential energy and gaining kinetic energy. This can be seen mathematically by the following:
[math]\displaystyle{ U_{ab} = -q \int_b^a \mathbf{E} \cdot d \mathbf{L} = - (q_n) (-E_o) \Delta L = (-\alpha q)E_o \Delta L = -\alpha q E_o \Delta L }[/math] (since the electric field is approximately constant between the plates & the electric field and path are aligned)
We can readily see that moving from position [math]\displaystyle{ b }[/math] to position [math]\displaystyle{ a }[/math] (a distance of length [math]\displaystyle{ \Delta L }[/math] ) causes a decrease in electric potential energy since this is a negative charge.
Now dividing [math]\displaystyle{ U_{ab} }[/math] by [math]\displaystyle{ q_n }[/math] gives [math]\displaystyle{ V_{ab} }[/math] :
[math]\displaystyle{ V_{ab} = \frac{U_{ab}}{q_n} = \frac{U_{ab}}{-\alpha q} = E_o \Delta L }[/math]
Therefore the electric potential is increasing.
- Question 3 :
We can reverse our answer from b. but keep in mind electric potential is per unit test charge ...essentially it is charge independent. Therefore, the electric potential energy of the positive charge would be increasing ( [math]\displaystyle{ \alpha q E_o \Delta L }[/math] ), and the electric potential would still be increasing.
A positive charge [math]\displaystyle{ q_o }[/math] travels through a spatially uniform electric field from Point [math]\displaystyle{ A }[/math] to Point [math]\displaystyle{ B }[/math] to Point [math]\displaystyle{ C }[/math] , was depicted in the figure below. The coordinates of the points and magnitude of the electric field are defined in the figure.
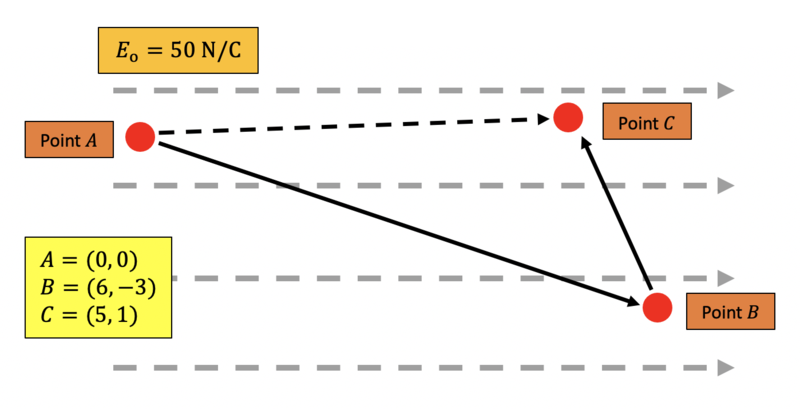
[math]\displaystyle{ V_{AB} = - \int_B^A \mathbf{E} \cdot d \mathbf{L} }[/math]
In this case, the electric field is a constant [math]\displaystyle{ (E_o, 0) }[/math] , and the path is a straight line with components: [math]\displaystyle{ \Delta \mathbf{L} = A-B = (0, 0) - (6, -3) = (-6, 3) }[/math]
Our expression for the change in electric potential simplifies to: [math]\displaystyle{ V_{AB} = -\mathbf{E} \cdot \Delta \mathbf{L} = -(E_o, 0) \cdot (-6, +3) = 6E_o = 6E_o = 300 \ \mathrm{V} }[/math]
The process is exactly the same as part a: [math]\displaystyle{ V_{BC} = -\int_C^B \mathbf{E} \cdot \Delta \mathbf{L} = - (E_o, 0) \cdot (1, -4) = -E_o = -50 \ \mathrm{V} }[/math]
We repeat the same process again: [math]\displaystyle{ V_{AC} = -\int_C^A \mathbf{E} \cdot \Delta \mathbf{L} = - (E_o, 0) \cdot (-5, 1) = 5E_o = 250 \ \mathrm{V} }[/math]
The three calculated electric potential differences are related by: [math]\displaystyle{ V_{AC} = V_{AB} + V_{BC} = 250 \ \mathrm{V} }[/math]
This illustrates the path independence of the potential difference, and thus the conservative nature of the electric field.
Connectedness
The concept of electric potential has a long, illustrious history that dates back many centuries. Otto von Guericke, William Gilbert, and Robert Boyle were among the pioneering scientists who contributed to the study of electricity in the 17th century. The idea of electric potential did not start to take form until the 18th century, however.
French scientist Charles Du Fay discovered in 1733 that friction could charge certain materials, including amber, and that this charge could be transmitted to other things. Du Fay also observed that depending on the sign of their charges, two charged items might either attract or repel one another when placed near to one another.
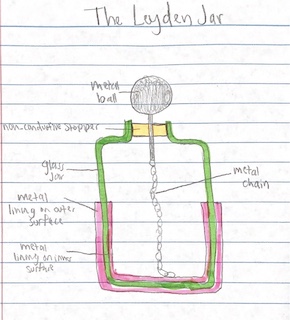
Both Dutch scientist Pieter van Musschenbroek and German physicist Ewald Georg von Kleist independently devised the Leyden jar, a device that could hold an electric charge, in 1745. The Leyden jar was simply a glass jar with a wire or rod put through the cork and either water or metal foil within. The charge would be kept in the jar until the wire was charged.
Charles-Augustin de Coulomb, a French scientist, first proposed the idea of electric potential energy in 1785. In an electric field, Coulomb demonstrated that the amount of labor needed to transfer a charged particle from one location to another was proportional to the difference in electric potential between the two sites. In the 19th century, Scottish scientist James Clerk Maxwell and German physicist Georg Simon Ohm expanded on this idea.
Electric potential is a key idea in physics today and is applied in a variety of domains, including electronics, electrical engineering, and the study of charged particle behavior in electromagnetic fields.
Further reading
Externals links.
Calculate the change in electric potential between point A, which is at [math]\displaystyle{ (-4, 3,0) \; m }[/math] , and B, which is at [math]\displaystyle{ (2,-2,0) \; m }[/math] . The electric field in the location is [math]\displaystyle{ (50,0,0) \; N/C }[/math] .
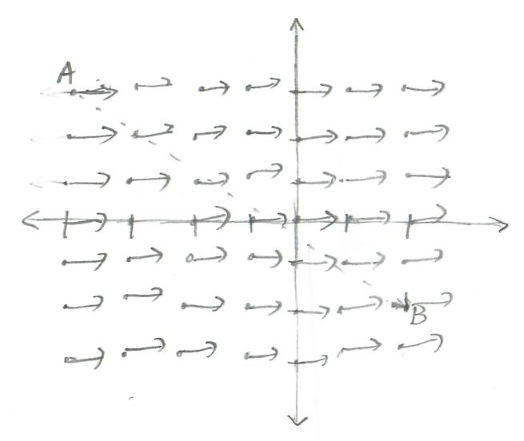
Answer: -300V
Explanation:
[math]\displaystyle{ \Delta\vec{l}= (2,-2,0)\;m - (-4,3,0)\;m = (6,-5,0)\;m }[/math]
[math]\displaystyle{ \Delta{V} = -({E}_{x}∆{x} + {E}_{y}∆{y} + {E}_{z}∆{z}) }[/math]
[math]\displaystyle{ \Delta{V} = -(50\; N/C \cdot 6 \;m + 0 \; N/c \cdot -5 \; m + 0\cdot 0) }[/math]
[math]\displaystyle{ \Delta{V} = -300 V }[/math]
The Bohr Model of the hydrogen atom treats it as a nucleus of charge [math]\displaystyle{ +e }[/math] electrons of charge [math]\displaystyle{ -e }[/math] orbiting in circular orbits of specific radii. Calculate the potential difference between two points, one infinitely far away from the nucleus, and the other one Bohr radius [math]\displaystyle{ a_0 }[/math] away from the nucleus (don't worry about substituting in the values). Compute the electric potential energy associated with the electron one Bohr radius away from the nucleus, setting the potential energy at infinity as zero (remember the sign of the charge).
We may compute the electric potential by taking integrating the electric field of a point charge as the radius goes from infinity to [math]\displaystyle{ a_0 }[/math] . This integral may be set up as:
[math]\displaystyle{ \Delta V = -\int_\infty^{a_0} \vec{E}\cdot\text{d}\vec{l} }[/math]
[math]\displaystyle{ \Delta V = -\frac{e}{4\pi\epsilon_0} \int_\infty^{a_0} \frac{\text{d} r}{r^2} }[/math]
This may then be solved:
[math]\displaystyle{ \Delta V = \frac{e}{4\pi\epsilon_0}\biggr{(}\frac{1}{r}\biggr{|}_\infty^{a_0} = \frac{e}{4\pi\epsilon_0 a_0} }[/math]
to compute the potential energy, we now multiply by the charge of the electron: [math]\displaystyle{ -e }[/math] , giving
[math]\displaystyle{ U = -\frac{e^2}{4\pi\epsilon_0 a_0} }[/math]
Depending on what physics or chemistry courses you may have taken before, this may be recognizable as twice the ionization energy of the n=1 orbital. The factor of two is due to the fact that in the Bohr model, the kinetic energy will be exactly half of this potential energy, and will be positive so that the net result is still negative, but with half the magnitude. This understanding of the hydrogen atom gets certain values right, but the reality of the situation is far more complicated.
How is this topic connected to something that you are interested in?
[Author] I am interested in robotic systems and building circuit boards and electrical systems for manufacturing robots. While studying this section in the book, I was able to connect back many of the concepts and calculations back to robotics and the electrical component of automated systems.
[Revisionist] Since high school, I never really understood how to work with the voltmeter and what it measured, and I have always wanted to know, but although this particular wiki page did not go into the details and other branches of electric potential, it led me to find the answers to something I was interested in since high school, the concept of electric potential.
[Editor] I think electively is really interesting. When I was younger, I participated in this demo where a group of people hold hands and someone touches this special ball full of charge. We all could feel the tingling sensation of the current passing through us. It’s cool to learn the theory behind the supposed magic that occurs.
[FALL 2018] I think it's very interesting that electric potential can be seen as a property of a space and that we can have further applications using this property.
How is it connected to your major?
[Author] I am a Mechanical Engineering major, so I will be dealing with the electrical components of machines when I work. Therefore, I have to know these certain concepts such as electric potential in order to fully understand how they work and interact.
[Revistionist] As a biochemistry major, electric potential and electric potential difference is not particularly related to my major, but in chemistry classes, we use electrostatic potential maps (electrostatic potential energy maps) that shows the charge distributions throughout a molecule. Although the main use in electric potential is different in physics and biochemistry (where physicists use it identify the effect of the electric field at a location), I still found it interesting as the concept of electric potential (buildup) was being used in quite a different way.
[Editor] I am a computer science major. Although I deal mostly with software, the hardware aspect is still important. The algorithms that I design run differently on different machines. The time complexity of an algorithm is sometimes useless when worrying about constant factors that are determined by a system’s hardware. Quicksort, for instance, is usually faster than many other sorts that have lower time complexities. The hardware of computers heavily relies on electricity and current (which is induced by a potential difference) to switch transistors on and off and thereby process information.
[FALL 2018] I am an aerospace engineering major, and I think understanding such concepts will help me have a better holistic understanding towards fields and systems. In addition, the thinking behind solving related problems will help me better prepared for future classes that involve with solving dynamics problems.
Is there an interesting industrial application?
[Author] Electrical potential is used to find the voltage across a path. This is useful when working with circuit components and attempting to manipulate the power output or current throughout a component.
[Revisionist] Electric potential sensors are being used to detect a variety of electrical signals made by the human body, thus contributing to the field of electrophysiology.
[Editor] The study of electric potential has lead scientists to generate very safe wires that will not overheat and cause fires. Connecting circuits to ground is important and the third prong in an electrical outlet is this ground connection.
The idea of electric potential, in a way, started with Ben Franklin and his experiments in the 1740s. He began to understand the flow of electricity, which eventually paved the path towards explaining electric potential and potential difference. Scientists finally began to understand how electric fields were actually affecting the charges and the surrounding environment. Benjamin Franklin first shocked himself in 1746, while conducting experiments on electricity with found objects from around his house. Six years later, or 261 years ago for us, the founding father flew a kite attached to a key and a silk ribbon in a thunderstorm and effectively trapped lightning in a jar. The experiment is now seen as a watershed moment in mankind's venture to channel a force of nature that was viewed quite abstractly.
By the time Franklin started experimenting with electricity, he'd already found fame and fortune as the author of Poor Richard's Almanack. Electricity wasn't a very well understood phenomenon at that point, so Franklin's research proved to be fairly foundational. The early experiments, experts believe, were inspired by other scientists' work and the shortcomings therein.
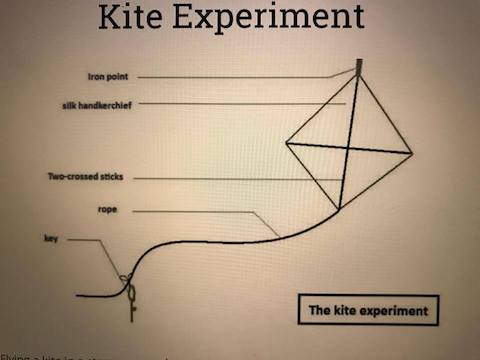
source: http://www.benjamin-franklin-history.org/kite-experiment/
That early brush with the dangers of electricity left an impression on Franklin. He described the sensation as "a universal blow throughout my whole body from head to foot, which seemed within as well as without; after which the first thing I took notice of was a violent quick shaking of my body." However, it didn't scare him away. In the handful of years before his famous kite experiment, Franklin contributed everything from designing the first battery designs to establishing some common nomenclature in the study of electricity. Although Franklin is often coined the father of electricity, after he set the foundations of electricity, many other scientists contributed his or her research in the advancement of electricity and eventually led to the discovery of electric potential and potential difference.
Like mentioned multiple times throughout the page, although electric potential is a huge and important topic, it has many branches, which makes the concept of electric potential difficult to stand alone. Even with this page, to support the concept of electric potential, many crucial branches of the topic appeared, like potential difference (which also branched into [ Potential Difference Path Independence ], [ Potential Difference In A Uniform Field ], and [ Potential Difference In A Nonuniform Field ]).
External Links
[1] https://www.khanacademy.org/test-prep/mcat/physical-processes/electrostatics-1/v/electric-potential-at-a-point-in-space
[2] https://www.youtube.com/watch?v=pcWz4tP_zUw
[3] https://www.youtube.com/watch?v=Vpa_uApmNoo
[4] https://www.khanacademy.org/science/electrical-engineering/ee-electrostatics/ee-fields-potential-voltage/a/ee-electric-potential-voltage
[1] "Benjamin Franklin and Electricity." Benjamin Franklin and Electricity. N.p., n.d. Web. 17 Apr. 2016. < http://www.americaslibrary.gov/aa/franklinb/aa_franklinb_electric_1.html >.
[2] Bottyan, Thomas. "Electrostatic Potential Maps." Chemwiki. N.p., 02 Oct. 2013. Web. 17 Apr. 2016. < http://chemwiki.ucdavis.edu/Core/Theoretical_Chemistry/Chemical_Bonding/General_Principles_of_Chemical_Bonding/Electrostatic_Potential_maps >.
[3] "Electric Potential Difference." Electric Potential Difference. The Physics Classroom, n.d. Web. 14 Apr. 2016. < http://www.physicsclassroom.com/class/circuits/Lesson-1/Electric-Potential-Difference >.
[4] Harland, C. J., T. D. Clark, and R. J. Prance. "Applications of Electric Potential (Displacement Current) Sensors in Human Body Electrophysiology." International Society for Industrial Process Tomography, n.d. Web. 16 Apr. 2016. < http://www.isipt.org/world-congress/3/269.html >.
[5] Sherwood, Bruce A. "2.1 The Momentum Principle." Matter & Interactions. By Ruth W. Chabay. 4th ed. Vol. 1. N.p.: John Wiley & Sons, 2015. 45-50. Print. Modern Mechanics.
Navigation menu
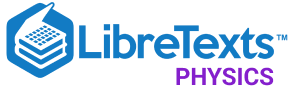
- school Campus Bookshelves
- menu_book Bookshelves
- perm_media Learning Objects
- login Login
- how_to_reg Request Instructor Account
- hub Instructor Commons
Margin Size
- Download Page (PDF)
- Download Full Book (PDF)
- Periodic Table
- Physics Constants
- Scientific Calculator
- Reference & Cite
- Tools expand_more
- Readability
selected template will load here
This action is not available.
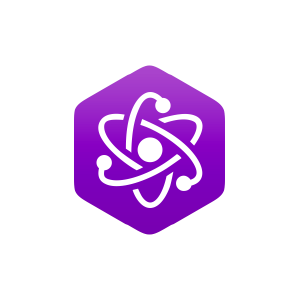
9.6: Electric Potential and Potential Energy
- Last updated
- Save as PDF
- Page ID 46934
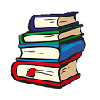
\( \newcommand{\vecs}[1]{\overset { \scriptstyle \rightharpoonup} {\mathbf{#1}} } \)
\( \newcommand{\vecd}[1]{\overset{-\!-\!\rightharpoonup}{\vphantom{a}\smash {#1}}} \)
\( \newcommand{\id}{\mathrm{id}}\) \( \newcommand{\Span}{\mathrm{span}}\)
( \newcommand{\kernel}{\mathrm{null}\,}\) \( \newcommand{\range}{\mathrm{range}\,}\)
\( \newcommand{\RealPart}{\mathrm{Re}}\) \( \newcommand{\ImaginaryPart}{\mathrm{Im}}\)
\( \newcommand{\Argument}{\mathrm{Arg}}\) \( \newcommand{\norm}[1]{\| #1 \|}\)
\( \newcommand{\inner}[2]{\langle #1, #2 \rangle}\)
\( \newcommand{\Span}{\mathrm{span}}\)
\( \newcommand{\id}{\mathrm{id}}\)
\( \newcommand{\kernel}{\mathrm{null}\,}\)
\( \newcommand{\range}{\mathrm{range}\,}\)
\( \newcommand{\RealPart}{\mathrm{Re}}\)
\( \newcommand{\ImaginaryPart}{\mathrm{Im}}\)
\( \newcommand{\Argument}{\mathrm{Arg}}\)
\( \newcommand{\norm}[1]{\| #1 \|}\)
\( \newcommand{\Span}{\mathrm{span}}\) \( \newcommand{\AA}{\unicode[.8,0]{x212B}}\)
\( \newcommand{\vectorA}[1]{\vec{#1}} % arrow\)
\( \newcommand{\vectorAt}[1]{\vec{\text{#1}}} % arrow\)
\( \newcommand{\vectorB}[1]{\overset { \scriptstyle \rightharpoonup} {\mathbf{#1}} } \)
\( \newcommand{\vectorC}[1]{\textbf{#1}} \)
\( \newcommand{\vectorD}[1]{\overrightarrow{#1}} \)
\( \newcommand{\vectorDt}[1]{\overrightarrow{\text{#1}}} \)
\( \newcommand{\vectE}[1]{\overset{-\!-\!\rightharpoonup}{\vphantom{a}\smash{\mathbf {#1}}}} \)
Learning Objectives
- Define electric potential and electric potential energy.
- Describe the relationship between electric potential difference and electric field.
- Describe the relationship between electric potential and electrical potential energy.
- Explain electron volt and its usage in submicroscopic process.
When a free positive charge \(q\) is accelerated by an electric field, such as shown in Figure \(\PageIndex{1}\), it is given kinetic energy. The process is analogous to an object being accelerated by a gravitational field. It is as if the charge is going down an electrical hill where its electric potential energy is converted to kinetic energy. Let us explore the work done on a charge \(q\) by the electric field in this process, so that we may develop a definition of electric potential energy.
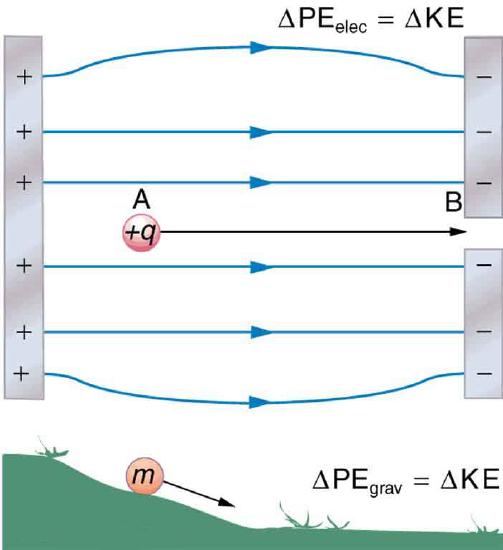
The electrostatic or Coulomb force is conservative, which means that the work done on \(q\) is independent of the path taken. This is exactly analogous to the gravitational force in the absence of dissipative forces such as friction. When a force is conservative, it is possible to define a potential energy associated with the force, and it is usually easier to deal with the potential energy (because it depends only on position) than to calculate the work done directly from force (\(W=\langle\boldsymbol{F}, \boldsymbol{d}\rangle\), where d is displacement and F is the force).
We use the letters PE to denote electric potential energy, which has units of joules (J). The change in potential energy, \(\triangle \mathrm{PE}\), is crucial, since the work done by a conservative force is the negative of the change in potential energy; that is, \(W=-\Delta \mathrm{PE}\). For example, work \(W\) done to accelerate a positive charge from rest is positive and results from a loss in PE, or a negative \(\triangle \mathrm{PE}\). There must be a minus sign in front of \(\triangle \mathrm{PE}\) to make \(W\) positive. PE can be found at any point by taking one point as a reference and calculating the work needed to move a charge to the other point.
Definition: POTENTIAL ENERGY
\(W=-\Delta \mathrm{PE}\). For example, work \(W\) done to accelerate a positive charge from rest is positive and results from a loss in PE, or a negative \(\Delta \mathrm{PE}\). There must be a minus sign in front of \(\Delta \mathrm{PE}\) to make \(W\) positive. PE can be found at any point by taking one point as a reference and calculating the work needed to move a charge to the other point.
Gravitational potential energy and electric potential energy are quite analogous. Potential energy accounts for work done by a conservative force and gives added insight regarding energy and energy transformation without the necessity of dealing with the force directly. It is much more common, for example, to use the concept of voltage (related to electric potential energy) than to deal with the electric field (related to Coulomb force) directly.
Given some conservative force \(F\) and displacement \(d\) under the force, the work done and the change in potential energy can be calculated as, \(W=\langle\boldsymbol{F}, \boldsymbol{d}\rangle\) and \(\Delta \mathrm{PE}=-\mathrm{W}=\langle-\mathbf{F}, \boldsymbol{d}\rangle\). For electric force, the force is given by the product of electric charge and the electric field, \(\boldsymbol{F}=q \boldsymbol{E}\), where \(q\) is the charge experiencing the force and \(E\) is the electric field at the location of the charge. So the potential energy change due to work done by electric force is \(\Delta \mathrm{PE}=q(\langle-\mathbf{E}, \boldsymbol{d}\rangle)\). If we define change in electric potential \(V\) as \(\Delta V=\langle-\mathbf{E}, \boldsymbol{d}\rangle\), then the electric potential energy PEPE is simply expressed in terms of electric potential, \(\mathrm{PE}=q V\), or,
\[V=\frac{\mathrm{PE}}{q}, \nonumber \]
electric potential energy per charge.
Definition: ELECTRIC POTENTIAL
Electric potential is the electric potential energy per unit charge.
\[V=\frac{\mathrm{PE}}{q} \nonumber\]
With potential energy, the case often is that its value at a single point has no significant meaning but what is important is the difference in potential energy. From the difference in potential energy, we are able to calculate other quantities, such as change in kinetic energy (if no force other than the conservative force acts) or work needing to be done by other forces (if other forces act). So likewise, rather than the electric potential itself, we are often interested in difference in electric potential \(\Delta V\) between two points, where,
\[\Delta V=V_{\mathrm{B}}-V_{\mathrm{A}}=\frac{\Delta \mathrm{PE}}{q}. \nonumber \]
The potential difference between points A and B, \(V_{\mathrm{B}}-V_{\mathrm{A}}\), is thus defined to be the change in potential energy of a charge \(q\) moved from A to B, divided by the charge. Units of potential difference are joules per coulomb, given the name volt (V) after Alessandro Volta.
\[1 \mathrm{~V}=1 \frac{\mathrm{J}}{\mathrm{C}} \nonumber \]
Definition: POTENTIAL DIFFERENCE
The potential difference between points A and B, \(V_{\mathrm{B}}-V_{\mathrm{A}}\), is defined to be the change in potential energy of a charge q moved from A to B, divided by the charge. Units of potential difference are joules per coulomb, given the name volt (V) after Alessandro Volta.
\[1 \mathrm{~V}=1 \frac{\mathrm{J}}{\mathrm{C}} \nonumber\]
The familiar term voltage is the common name for potential difference. Keep in mind that whenever a voltage is quoted, it is understood to be the potential difference between two points. For example, every battery has two terminals, and its voltage is the potential difference between them. More fundamentally, the point you choose to be zero volts is arbitrary. This is analogous to the fact that gravitational potential energy has an arbitrary zero, such as sea level or perhaps a lecture hall floor.
In summary, the relationship between potential difference (or voltage) and electrical potential energy is given by
\[\Delta V=\frac{\Delta \mathrm{PE}}{q} \text { and } \Delta \mathrm{PE}=q \Delta V. \nonumber \]
POTENTIAL DIFFERENCE AND ELECTRICAL POTENTIAL ENERGY
The relationship between potential difference (or voltage) and electrical potential energy is given by
\[\Delta V=\frac{\Delta \mathrm{PE}}{q} \text { and } \Delta \mathrm{PE}=q \Delta V. \nonumber\]
The second equation is equivalent to the first.
Voltage is not the same as energy. Voltage is the energy per unit charge. Thus a motorcycle battery and a car battery can both have the same voltage (more precisely, the same potential difference between battery terminals), yet one stores much more energy than the other since \(\Delta \mathrm{PE}=q \Delta V\). The car battery can move more charge than the motorcycle battery, although both are 12 V batteries.
Example \(\PageIndex{1}\): Calculating Energy
Suppose you have a 12.0 V motorcycle battery that can move 5000 C of charge, and a 12.0 V car battery that can move 60,000 C of charge. How much energy does each deliver? (Assume that the numerical value of each charge is accurate to three significant figures.)
To say we have a 12.0 V battery means that its terminals have a 12.0 V potential difference. When such a battery moves charge, it puts the charge through a potential difference of 12.0 V, and the charge is given a change in potential energy equal to \(\Delta \mathrm{PE}=q \Delta V\).
So to find the energy output, we multiply the charge moved by the potential difference.
For the motorcycle battery, \(q=5000 \ \mathrm{C}\) and \(\Delta V=12.0 \mathrm{~V}\). The total energy delivered by the motorcycle battery is
\[\begin{aligned} \Delta \mathrm{PE}_{\text {cycle }} &=(5000 \mathrm{C})(12.0 \mathrm{~V}) \\ &=(5000 \mathrm{C})(12.0 \mathrm{~J} / \mathrm{C}) \\ &=6.00 \times 10^{4} \mathrm{~J}. \end{aligned} \nonumber\]
Similarly, for the car battery, \(q=60,000 \ \mathrm{C}\) and
\[\begin{aligned} \Delta \mathrm{PE}_{\mathrm{car}} &=(60,000 \mathrm{C})(12.0 \mathrm{~V}) \\ &=7.20 \times 10^{5} \mathrm{~J}. \end{aligned} \nonumber\]
While voltage and energy are related, they are not the same thing. The voltages of the batteries are identical, but the energy supplied by each is quite different. Note also that as a battery is discharged, some of its energy is used internally and its terminal voltage drops, such as when headlights dim because of a low car battery. The energy supplied by the battery is still calculated as in this example, but not all of the energy is available for external use.
Note that the energies calculated in the previous example are absolute values. The change in potential energy for the battery is negative, since it loses energy. These batteries, like many electrical systems, actually move negative charge—electrons in particular. The batteries repel electrons from their negative terminals (A) through whatever circuitry is involved and attract them to their positive terminals (B) as shown in Figure \(\PageIndex{2}\). The change in potential is \(\Delta V=V_{\mathrm{B}}-V_{\mathrm{A}}=+12 \mathrm{~V}\) and the charge \(q\) is negative, so that \(\Delta \mathrm{PE}=q \Delta V\) is negative, meaning the potential energy of the battery has decreased when \(q\) has moved from A to B.
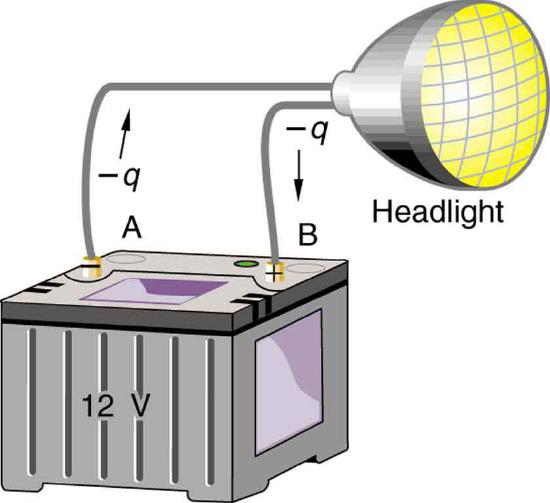
Example \(\PageIndex{2}\): How Many Electrons Move through a Headlight Each Second?
When a 12.0 V car battery runs a single 30.0 \(W\) headlight, how many electrons pass through it each second?
To find the number of electrons, we must first find the charge that moved in 1.00 s. The charge moved is related to voltage and energy through the equation \(\Delta \mathrm{PE}=q \Delta V\). A 30.0 W lamp uses 30.0 joules per second. Since the battery loses energy, we have \(\Delta \mathrm{PE}=-30.0 \mathrm{~J}\) and, since the electrons are going from the negative terminal to the positive, we see that \(\Delta V=+12.0 \mathrm{~V}\).
To find the charge \(q\) moved, we solve the equation \(\Delta \mathrm{PE}=q \Delta V\):
\[q=\frac{\Delta \mathrm{PE}}{\Delta V}. \nonumber\]
Entering the values for \(\triangle \mathrm{PE}\) and \(\Delta V\), we get
\[q=\frac{-30.0 \mathrm{~J}}{+12.0 \mathrm{~V}}=\frac{-30.0 \mathrm{~J}}{+12.0 \mathrm{~J} / \mathrm{C}}=-2.50 \mathrm{C}. \nonumber\]
The number of electrons \(\mathrm{n}_{\mathrm{e}}\) is the total charge divided by the charge per electron. That is,
\[\mathrm{n}_{\mathrm{e}}=\frac{-2.50 \mathrm{C}}{-1.60 \times 10^{-19} \mathrm{C} / \mathrm{e}^{-}}=1.56 \times 10^{19} \text { electrons. } \nonumber\]
This is a very large number. It is no wonder that we do not ordinarily observe individual electrons with so many being present in ordinary systems. In fact, electricity had been in use for many decades before it was determined that the moving charges in many circumstances were negative. Positive charge moving in the opposite direction of negative charge often produces identical effects; this makes it difficult to determine which is moving or whether both are moving.
The Electron Volt
The energy per electron is very small in macroscopic situations like that in the previous example—a tiny fraction of a joule. But on a submicroscopic scale, such energy per particle (electron, proton, or ion) can be of great importance. For example, even a tiny fraction of a joule can be great enough for these particles to destroy organic molecules and harm living tissue. The particle may do its damage by direct collision, or it may create harmful X-rays, which can also inflict damage. It is useful to have an energy unit related to submicroscopic effects. Figure \(\PageIndex{3}\) shows a situation related to the definition of such an energy unit. An electron is accelerated between two charged metal plates as it might be in an old-model television tube or oscilloscope. The electron is given kinetic energy that is later converted to another form—light in the television tube, for example. (Note that downhill for the electron is uphill for a positive charge.) Since energy is related to voltage by \(\Delta \mathrm{PE}=q \Delta V\), we can think of the joule as a coulomb-volt.
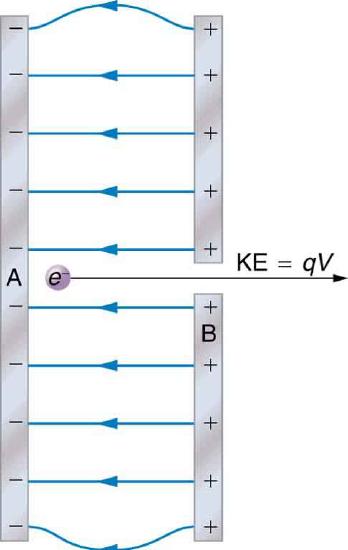
Definition: ELECTRON VOLT
On the submicroscopic scale, it is more convenient to define an energy unit called the electron volt (eV), which is the energy given to a fundamental charge accelerated through a potential difference of 1 V . In equation form,
\[\begin{aligned} 1 \mathrm{eV} &=\left(1.60 \times 10^{-19} \mathrm{C}\right)(1 \mathrm{~V})=\left(1.60 \times 10^{-19} \mathrm{C}\right)(1 \mathrm{~J} / \mathrm{C}) \\ &=1.60 \times 10^{-19} \mathrm{~J}. \end{aligned} \nonumber\]
An electron accelerated through a potential difference of 1 V is given an energy of 1 eV. It follows that an electron accelerated through 50 V is given 50 eV. A potential difference of 100,000 V (100 kV) will give an electron an energy of 100,000 eV (100 keV), and so on. Similarly, an ion with a double positive charge accelerated through 100 V will be given 200 eV of energy. These simple relationships between accelerating voltage and particle charges make the electron volt a simple and convenient energy unit in such circumstances.
CONNECTIONS: ENERGY UNITS
The electron volt (eV) is the most common energy unit for submicroscopic processes. This will be particularly noticeable in the chapters on modern physics. Energy is so important to so many subjects that there is a tendency to define a special energy unit for each major topic. There are, for example, calories for food energy, and kilowatt-hours for electrical energy.
The electron volt is commonly employed in submicroscopic processes—chemical valence energies and molecular and nuclear binding energies are among the quantities often expressed in electron volts. For example, about 5 eV of energy is required to break up certain organic molecules. If a proton is accelerated from rest through a potential difference of 30 kV, it is given an energy of 30 keV (30,000 eV) and it can break up as many as 6000 of these molecules (\(30,000 \ \mathrm{eV} \div 5 \mathrm{eV} \text { per molecule }=6000 \text { molecules }\)). Nuclear decay energies are on the order of 1 MeV (1,000,000 eV) per event and can, thus, produce significant biological damage.
Conservation of Energy
The total energy of a system is conserved if there is no net addition (or subtraction) of work or heat transfer. For conservative forces, such as the electrostatic force, conservation of energy states that mechanical energy is a constant.
Mechanical energy is the sum of the kinetic energy and potential energy of a system; that is, \(\mathrm{KE}+\mathrm{PE}=\text { constant }\). A loss of PE of a charged particle becomes an increase in its KE. Here PE is the electric potential energy. Conservation of energy is stated in equation form as
\[\mathrm{KE}+\mathrm{PE}=\text { constant } \nonumber \]
\[\mathrm{KE}_{\mathrm{i}}+\mathrm{PE}_{\mathrm{i}}=\mathrm{KE}_{\mathrm{f}}+\mathrm{PE}_{\mathrm{f}}, \nonumber \]
where i and f stand for initial and final conditions. As we have found many times before, considering energy can give us insights and facilitate problem solving.
Example \(\PageIndex{3}\): Electrical Potential Energy Converted to Kinetic Energy
Calculate the final speed of a free electron accelerated from rest through a potential difference of 100 V. (Assume that this numerical value is accurate to three significant figures.)
We have a system with only conservative forces. Assuming the electron is accelerated in a vacuum, and neglecting the gravitational force (we will check on this assumption later), all of the electrical potential energy is converted into kinetic energy. We can identify the initial and final forms of energy to be \(\mathrm{KE}_{\mathrm{i}}=0, \mathrm{KE}_{\mathrm{f}}=1 / 2 m v^{2}, \mathrm{PE}_{\mathrm{i}}=q V, \text { and } \mathrm{PE}_{\mathrm{f}}=0\).
Conservation of energy states that
\[\mathrm{KE}_{\mathrm{i}}+\mathrm{PE}_{\mathrm{i}}=\mathrm{KE}_{\mathrm{f}}+\mathrm{PE}_{\mathrm{f}}. \nonumber\]
Entering the forms identified above, we obtain
\[q V=\frac{m v^{2}}{2}. \nonumber\]
We solve this for \(v\) :
\[v=\sqrt{\frac{2 q V}{m}}. \nonumber\]
Entering values for \(q\),\(V\), and \(m\) gives
\[\begin{aligned} v &=\sqrt{\frac{2\left(-1.60 \times 10^{-19} \mathrm{C}\right)(-100 \mathrm{~J} / \mathrm{C})}{9.11 \times 10^{-31} \mathrm{~kg}}} \\ &=5.93 \times 10^{6} \mathrm{~m} / \mathrm{s}. \end{aligned} \nonumber\]
Note that both the charge and the initial voltage are negative, as in Figure \(\PageIndex{3}\). We know that electrostatic forces on small particles are generally very large compared with the gravitational force. The large final speed confirms that the gravitational force is indeed negligible here. The large speed also indicates how easy it is to accelerate electrons with small voltages because of their very small mass. Voltages much higher than the 100 V in this problem are typically used in electron guns.
Section Summary
- Electric potential is potential energy per unit charge.
\[.\Delta V=\frac{\Delta \mathrm{PE}}{q} \text { and } \Delta \mathrm{PE}=q \Delta V \nonumber\]
- Mechanical energy is the sum of the kinetic energy and potential energy of a system, that is, \(\mathrm{KE}+\mathrm{PE}\) This sum is a constant.
Nature's Electric Potential: A Systematic Review of the Role of Bioelectricity in Wound Healing and Regenerative Processes in Animals, Humans, and Plants
Affiliation.
- 1 John Ray Research Field StationCheshire, United Kingdom.
- PMID: 28928669
- PMCID: PMC5591378
- DOI: 10.3389/fphys.2017.00627
Natural endogenous voltage gradients not only predict and correlate with growth and development but also drive wound healing and regeneration processes. This review summarizes the existing literature for the nature, sources, and transmission of information-bearing bioelectric signals involved in controlling wound healing and regeneration in animals, humans, and plants. It emerges that some bioelectric characteristics occur ubiquitously in a range of animal and plant species. However, the limits of similarities are probed to give a realistic assessment of future areas to be explored. Major gaps remain in our knowledge of the mechanistic basis for these processes, on which regenerative therapies ultimately depend. In relation to this, it is concluded that the mapping of voltage patterns and the processes generating them is a promising future research focus, to probe three aspects: the role of wound/regeneration currents in relation to morphology; the role of endogenous flux changes in driving wound healing and regeneration; and the mapping of patterns in organisms of extreme longevity, in contrast with the aberrant voltage patterns underlying impaired healing, to inform interventions aimed at restoring them.
Keywords: Vmem; electric field; regeneration; voltage mapping; wound current.
Publication types
Accessibility Links
- Skip to content
- Skip to search IOPscience
- Skip to Journals list
- Accessibility help
- Accessibility Help
Click here to close this panel.
Lunar surface potential and electric field *
Lei Li 1 , Yi-Teng Zhang 1,2 , Bin Zhou 1 and Yong-Yong Feng 1
© 2019 National Astronomical Observatories, CAS and IOP Publishing Ltd. Research in Astronomy and Astrophysics , Volume 19 , Number 6 Citation Lei Li et al 2019 Res. Astron. Astrophys. 19 077 DOI 10.1088/1674-4527/19/6/77
Article metrics
844 Total downloads
Permissions
Get permission to re-use this article
Share this article
Author e-mails.
Author affiliations
1 State Key Laboratory for Space Weather, National Space Science Center, Chinese Academy of Sciences, Beijing 100190, China
2 University of Chinese Academy of Sciences, Beijing 100049, China
- Received 19 October 2018
- Accepted 2 April 2019
The Moon has no significant atmosphere, thus its surface is exposed to solar ultraviolet radiation and the solar wind. Photoemission and collection of the solar wind electrons and ions may result in lunar surface charging. On the dayside, the surface potential is mainly determined by photoelectrons, modulated by the solar wind; while the nightside surface potential is a function of the plasma distribution in the lunar wake. Taking the plasma observations in the lunar environment as inputs, the global potential distribution is calculated according to the plasma sheath theory, assuming Maxwellian distributions for the surface emitted photoelectrons and the solar wind electrons. Results show that the lunar surface potential and sheath scale length change versus the solar zenith angle, which implies that the electric field has a horizontal component in addition to the vertical one. By differentiating the potential vertically and horizontally, we obtain the global electric field. It is found that the vertical electric field component is strongest at the subsolar point, which has a magnitude of 1 V m −1 . The horizontal component is much weaker, and mainly appears near the terminator and on the nightside, with a magnitude of several mV m −1 . The horizontal electric field component on the nightside is rotationally symmetric around the wake axis and is strongly determined by the plasma parameters in the lunar wake.
Export citation and abstract BibTeX RIS
1. Introduction
Celestial bodies exposed to the space environment will accumulate charge due to the incident solar radiation and space plasma, resulting in an inhomogeneous plasma sheath around the body. Previous research has shown that the equilibrium potential of a celestial body can be determined by balancing all charging currents, and among them transfer of charge from the ambient plasma, photo-electron emission and secondary electron emission of the surface are most important. Moreover, all currents are determined by the surface characteristics and environmental conditions. Without photoemission, the incident electron flux from the ambient plasma is usually larger than the ion flux, and the equilibrium surface potential will have a negative value, with the potential in V on the same order of magnitude as the plasma electron energy in eV. However, if the photoelectron emission dominates, the equilibriumsurface potential will have a positive value on the same order of magnitude as the photoelectron energy in eV (Whipple 1981 ).
The lunar exosphere is extremely tenuous, thus it is usually negligible. As a result, the lunar surface is exposed to solar radiation, the solar wind or the Earth's magnetosphere plasma. The incident ambient plasma injection, photoelectrons and secondary electrons emitted by the lunar regolith can all cause charging of the lunar surface. From the ion measurements on the lunar surface by Apollo 14, Freeman & Ibrahim ( 1975 ) deduced that the surface potential is a function of the solar zenith angle ( sza ), with the highest potential at the subsolar point ( sza = 0), which is about +10 V. The surface potential declines with sza . At the terminator, the potential drops to about −100 V. From the electron reflectometry of Lunar Prospector (Halekas et al. 2002 ), the potential difference between the nightside lunar surface and the spacecraft is found to be −35 V, and hence the scale thickness of the electric double layer is 1 ∼ 2 km on the nightside. However since the potential of the spacecraft is unknown, the lunar surface potential on the nightside cannot be deduced. The surface charging is a result of several coupled mechanisms, and the electric condition on the surface should be much more complicated than the scenario depicted by such limited measurements.
Classic plasma sheath theory can help in understanding the lunar surface charging and surface electric field. By solving Poisson's equation, the potential and electric field inside the sheath, as well as their variations with sza , can be calculated (Manka 1973 ). In recent years, a Particle-In-Cell (PIC) simulation has been applied to the lunar sheath. Through one dimensional (1D) PIC simulation, the effects of the non-Maxwellian distribution of photoelectrons, and the solar radiation variation on the lunar sheath were investigated (Poppe & Horányi 2010 ). PIC can also be extended to the three dimensional (3D) context to simulate the local electric field resulting from small scale lunar topology (Anuar et al. 2013 ).
Due to uncertainty about the plasma environment inside the lunar wake, previous simulations of the lunar sheath and surface electric field are usually limited to the dayside, where the reflection of the solar wind by the lunar surface is not considered, and the solar wind is treated as undisturbed at the upper boundary of the lunar sheath. Our knowledge about the ambient plasma around theMoon mainly comes from spacecraft observations at altitudes of tens kilometers above the lunar surface. Results show that, on the nightside, the ambient plasma is quite different from the undisturbed solar wind upstream of the Moon (Halekas et al. 2005 ).
Both observations and theoretical works agreed that the surface potential is a function of sza (Freeman & Ibrahim 1975 ; Manka 1973 ), since both the solar radiation and solar wind change with sza . It follows naturally that a large scale horizontal electric field exists on the Moon in addition to the vertical electric field, especially at the dawn-dusk region, where the solar radiation and solar wind conditions change dramatically. In this paper, based on the lunar plasma observations in recent years, based on a 1D sheath model (Lei et al. 2016 ), potential distributions at different sza are analyzed and a global 3D electric field distribution is deduced when the Moon is submerged in the solar wind.
2. Lunar surface sheath model
In the solar wind, the Debye length ( λ d ) is much smaller than the radius of the Moon ( R m ). Ignoring local geological features, a 1D model is suitable to describe the plasma sheath over the surface of the Moon (Nitter et al. 1998 ). Supposing x is perpendicular to the lunar surface, the angle between x and the Moon-Sun line is the sza . Ignoring aberration of the solar wind, the solar radiation and solar wind impact the lunar surface along the same direction, which makes an angle of sza with respect to x . The lunar regolith emits photoelectrons under the impact of solar UV. Moreover, the incoming solar wind mostly has low energy, and the secondary electrons emitted by the lunar regolith resulting from bombardment of the solar wind can be neglected (Whipple 1981 ).
At thermodynamic equilibrium, the potential V and charged particle density n in the sheath are governed by Poisson's equation,
where subscripts i , e and ph represent the solar wind proton, solar wind electron and photoelectron emitted by the lunar surface respectively; e is the elementary charge and ε 0 is the vacuum permittivity.
The motion of charged particles satisfies the continuity and energy conservation equations, ignoring gravity, which can be written as
where m , v , q and x are particle mass, velocity, charge and position respectively; q = e for protons, q = − e for electrons; V is the electric potential at x ; the subscript 0 denotes the initial state. Usually, the thermal velocity of a proton is much lower than its bulk velocity, so v in Equation ( 1 ) is approximately equal to the proton bulk velocity; on the contrary, the electron thermal velocity is much higher than its bulk velocity, and v in Equation ( 1 ) is taken as its thermal velocity.
It is assumed that both solar wind electrons and the photoelectrons emitted by the lunar surface are Maxwellian. The distribution function can be written as
where m e is the electron mass, n j is the number density, T j is the electron temperature, k is the Boltzmann constant, j = e for solar wind electrons and j = ph for photoelectrons. Integration of Equation ( 2 ) in the velocity space yields the electron density n e and n ph at x . Since the proton is mono-energetic, n i can be obtained through energy conservation and the continuity equation,
where subscript 0 represents the incoming solar wind parameters.
Combining Equations ( 2 ), ( 3 ) and ( 4 ), together with the quasi-neutrality and zero net current conditions at infinity, a steady state sheath model can be established to determine the potential distribution inside the sheath, which is expected to depend on the solar radiation and solar wind conditions.
3. Sheath profile at different sza
3.1. solar wind conditions near the moon.
Early studies believed that the solar wind plasma is absorbed by the lunar surface, leading to the formation of a plasma wake behind the Moon, meanwhile the interplanetarymagnetic field (IMF) passes through theMoonwithout significant distortion in its direction or magnitude.
The characteristics of solar wind electrons and the magnetic field near the Moon are well understood thanks to Lunar Prospector's long term observations at altitudes of several tens of kilometers. Halekas et al. ( 2005 ) give the normalized solar wind electron and temperature binned by the angle from the wake axis, which is aligned with the solar wind flow direction. Since we ignore the solar wind aberration here, the angle from the wake axis coincides with sza . The solar wind electron density and temperature stay largely undisturbed on the dayside. At sza > 90°, the density decreases monotonically to a minimum at the center of the wake, while the temperature increases first, shows a high level of variability around sza = 150°, and then decreases towards the center of the wake (Fig. 1 ). Here we should note that although the electron temperature inside the wake might be several times that of the undisturbed solar wind, it is still too low to produce significant secondary electron emission when the solar wind electrons impact on the lunar surface (Whipple 1981 ).
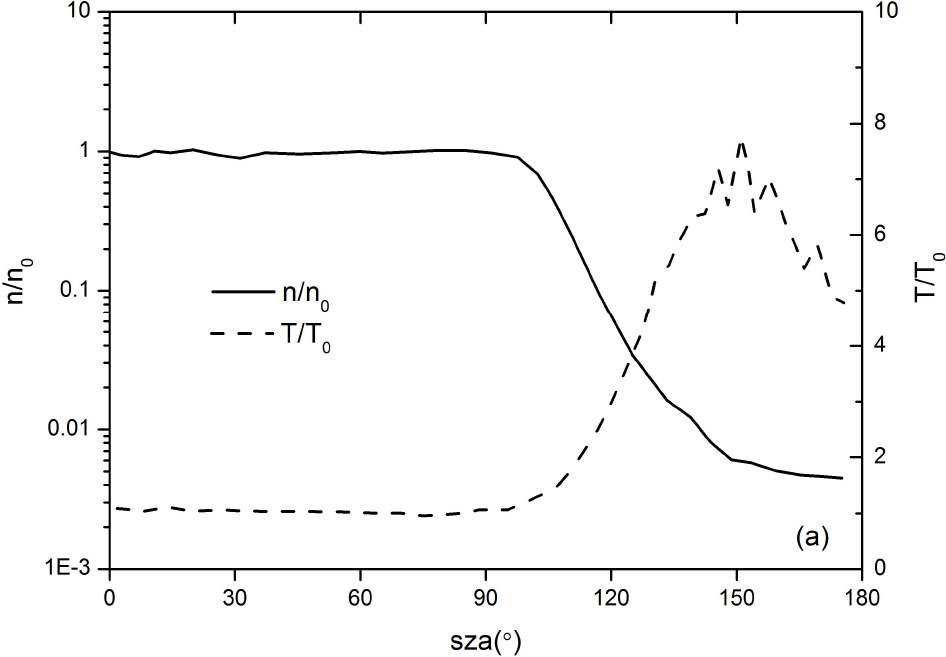
Fig. 1 Normalized solar wind electron density and temperature versus sza in the quasi-perpendicular wake regions, adapted from Halekas et al. ( 2005 ).
Download figure:
However, the proton behavior is not so well known in the wake. With higher thermal velocity, the electrons refill the wake along the magnetic field lines much more quickly Fig. 1 Normalized solar wind electron density and temperature versus sza in the quasi-perpendicular wake regions, adapted from Halekas et al. ( 2005 ). than the ions. So for a long time, it was believed that the near wake is a negatively charged region. The scale height of the electric double layer in the near wake can be as large as 200 km (Borisov & Mall 2002 ). However, recent observations suggest that the ambipolar electric field at the lunar wake boundarymay accelerate the solar wind protons deep into the lunar wake (Nishino et al. 2009 ). Furthermore, the solar wind particles impacting on the dayside are not totally absorbed by lunar regolith, with 1% or more protons reflected or scattered by the lunar surface (Saito et al. 2008 ); meanwhile, the lunar regolith may also be sputtered by the solar wind. All the charged particles, which are reflected, scattered and sputtered, may be accelerated by the solar wind electric field, moving around the Moon into the near wake (Nishino et al. 2009 ; Wang et al. 2010 ). It can be inferred from these observations that the near wake is not a negatively charged region, but a region with quasineutrality (Nishino et al. 2009 ).
Based on these observations, it seems reasonable to assume that the protons and electrons have the same density to keep quasi-neutrality in the near wake. We also assume that the protons have the same temperature as the electrons. Because the proton velocity is much lower than the electron thermal velocity, its effects on the sheath are relatively minor. The uncertainty in the temperature might cause error in the sheath potential, but no significant influence is expected.
In the following calculation, the undisturbed solar wind parameters on the dayside are v 0 = 400 km s −1 , n 0 = 5 cm −3 and T e = 15 eV, while the nightside parameters follow LP's observations (Fig. 1 ). The solar wind temperature in the wake is fitted with a Gaussian function centered at sza = 150°. The temperature of a photoelectron emitted by the lunar regolith is 1.47 eV, and the photoelectron current density is 4.5 × 10 −6 cos( sza ) A m −2 (Nitter et al. 1998 ). The photoelectron emission is cut off when sza ≥ 90°.
3.2. Sheath Profiles vs. sza
3.2.1. dayside sheath.
On the dayside, the photoelectron current density, as well as the solar wind proton normal incident flux, depends on sza . The sheath potential profiles for sza = 0°, 50°, 80° and 90° are shown in Figure 2 . As expected, the sheath potential profile changes significantly with sza . When sza is low, photoelectron emission is high. Near the surface, the photoelectrons have a much higher concentration than the solar wind particles (see Fig. 3 , sza = 0°), so the sheath is also called a "photoelectron sheath." The equilibrium surface potential is positive. Accumulation of photoelectrons inside the sheath leads to a potential trap as seen from the non-monotonic potential profile. The photoelectron emission and normal incident proton flux decrease with increasing sza . At sza = 80°, the entire sheath, including the surface, has a negative potential. At sza = 90°, there is no photoelectron emission, but the solar wind electron with high thermal velocity can still impact the surface to build up a negative potential (Fig. 2 , sza = 90°). At the same time, the proton can still move into the sheath with the thermal velocity although its normal bulk velocity is 0. Consequently, we set the minimum velocity of the proton as the ion acoustic velocity (Nitter et al. 1998 ). Otherwise without positive ion charge, no stable sheath can form in front of a negatively charged absorbing surface (Riemann 1991 ). Under the attraction of the negatively charged surface, protons are gathered around the surface (Fig. 3 , sza = 90°), forming an ion sheath, screening the effect of negative surface potential within a distance of about 100 m.
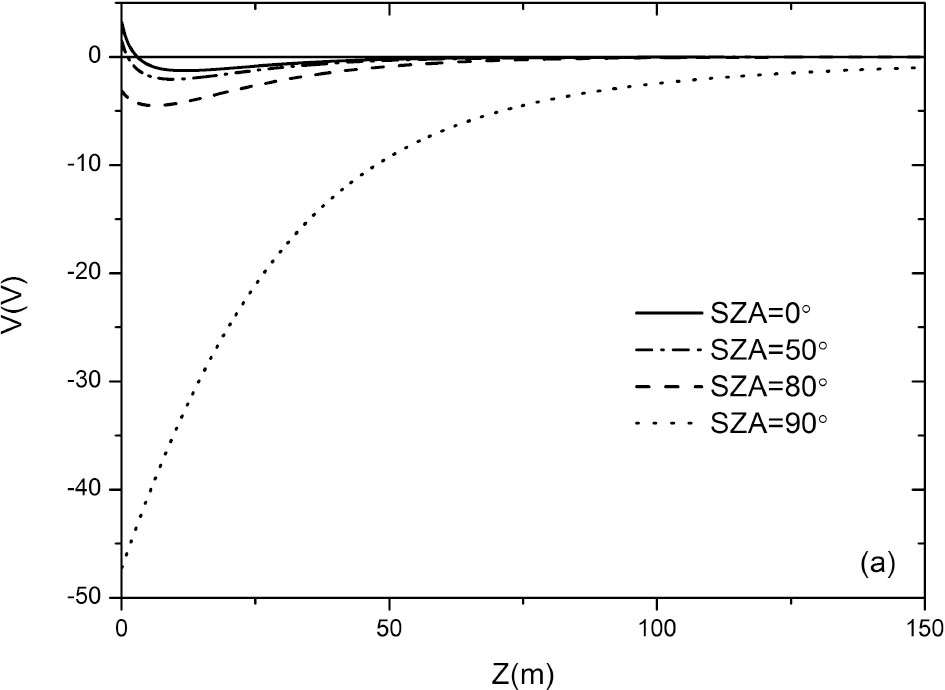
Fig. 2 Sheath potential profiles from the subsolar ( sza = 0°) to the terminator on the dayside, for sza = 0°, 50°, 80° and 90°.
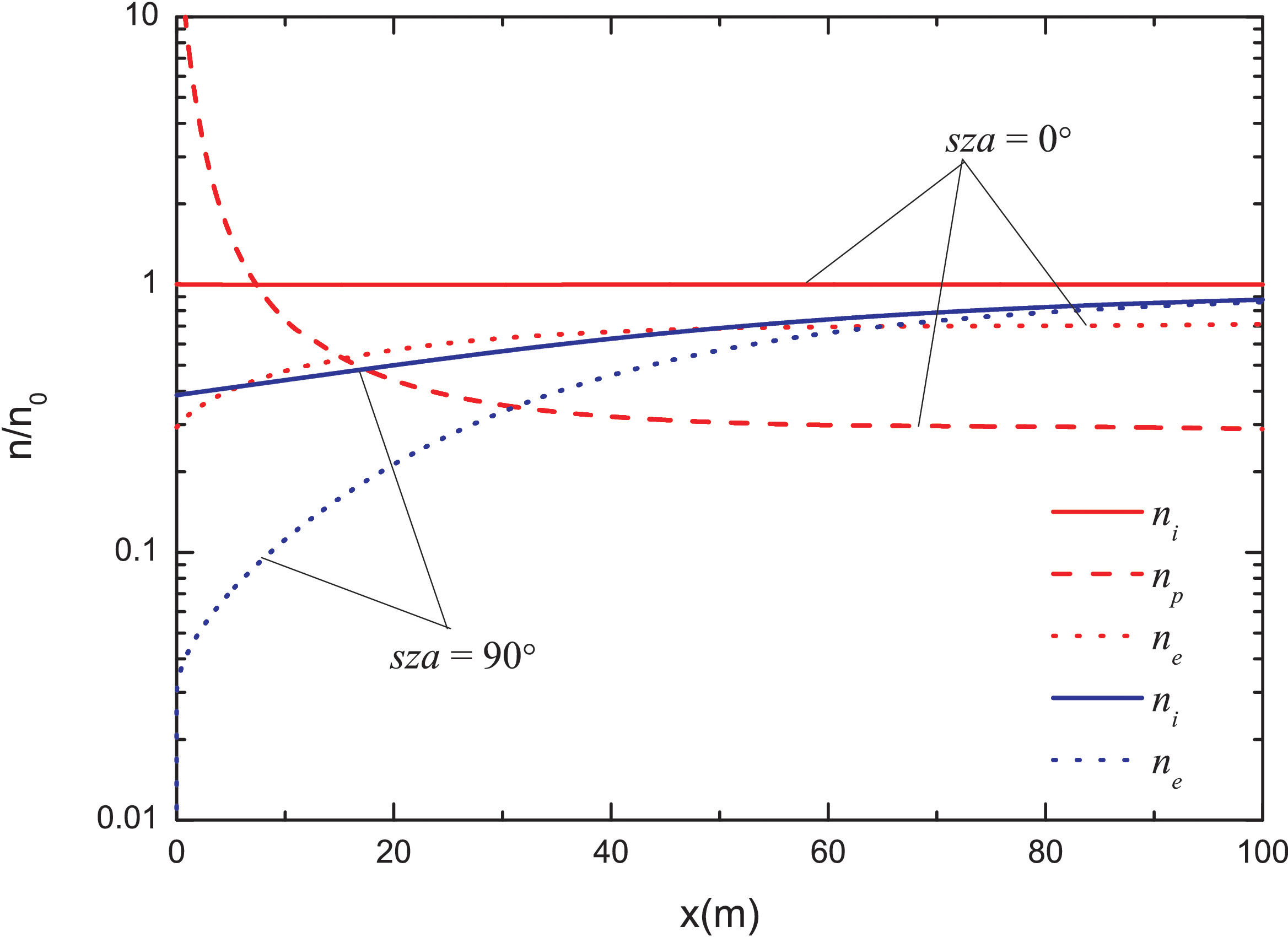
Fig. 3 Charged particle densities in the sheath when sza = 0° or 90° ( Color version is online) .
In a photoelectron sheath ( sza = 0°), photoelectrons dominate near the surface. The proton density stays almost constant throughout the sheath. The proton serves as a component in current balance, especially far away from the surface where the proton and electron density are nearly equal. On the contrary, in an ion sheath ( sza = 90°), the proton density is higher than the electron density in the whole sheath.
3.2.2. Nightside sheath
When sza is above 90°, although there is no surface photoemission, and the plasma density inside the wake is very low, the lunar surface can still accumulate charges due to its low conductivity (Borisov & Mall 2002 ). Referring to the result of particle simulation (Ye 2010 ), we assume that a proton in the wake has no drift velocity toward the surface, but can reach the surface with the ion acoustic velocity to maintain a stable sheath.
As expected, the surface potential stays negative due to the higher mobility of electrons in the wake. The surface potential decreases with sza as the electron temperature increases in the wake, and reaches a minimum value of −300 V at sza = 150° (Fig. 4 ), but increases when sza > 150°. At the center of the wake ( sza = 180°), both the electron temperature and density are lower, and the surface potential is higher than that at sza = 150°. Figure 4 suggests that the electron temperature inside the wake is a dominant factor in determining the surface potential on the nightside. According to Figure 4 , the thickness of the sheath is more than several hundred meters on the nightside. Our result is close to the observation results by LP (Halekas et al. 2003 ), but much smaller than that estimated by the model which assumes that the near wake is negatively charged with an electric double layer as thick as 200 km (Borisov & Mall 2002 ).
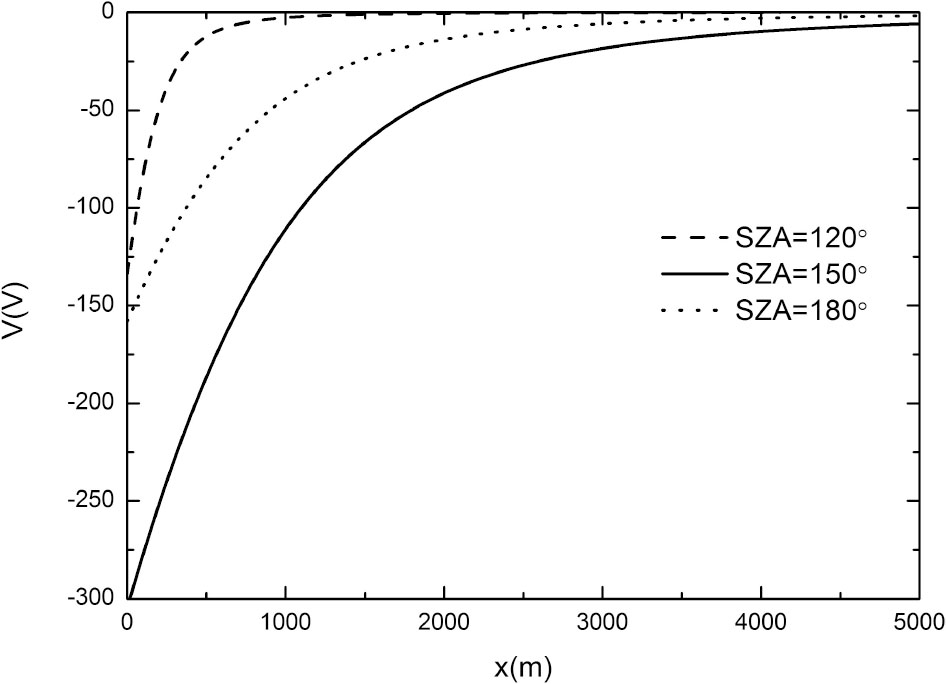
Fig. 4 Sheath potential profiles on nightside.

3.3. Global Electric Field
The changes in the surface potential and Debye length vs. sza are summarized in Figure 5 . At sza ≤ 80°, the surface potential changes by several volts from positive to negative; while the Debye length stays around 1 m. Around the terminator ( sza = 90°), as the solar wind parameters change abruptly with sza , the surface potential drops while the Debye length increases sharply. On the nightside, the surface potential can reach negative several hundred volts, and the Debye length can be as large as several hundred meters (Fig. 5 ). The slope discontinuity at sza = 90° is caused by the abrupt cutoff of solar radiation at the terminator.
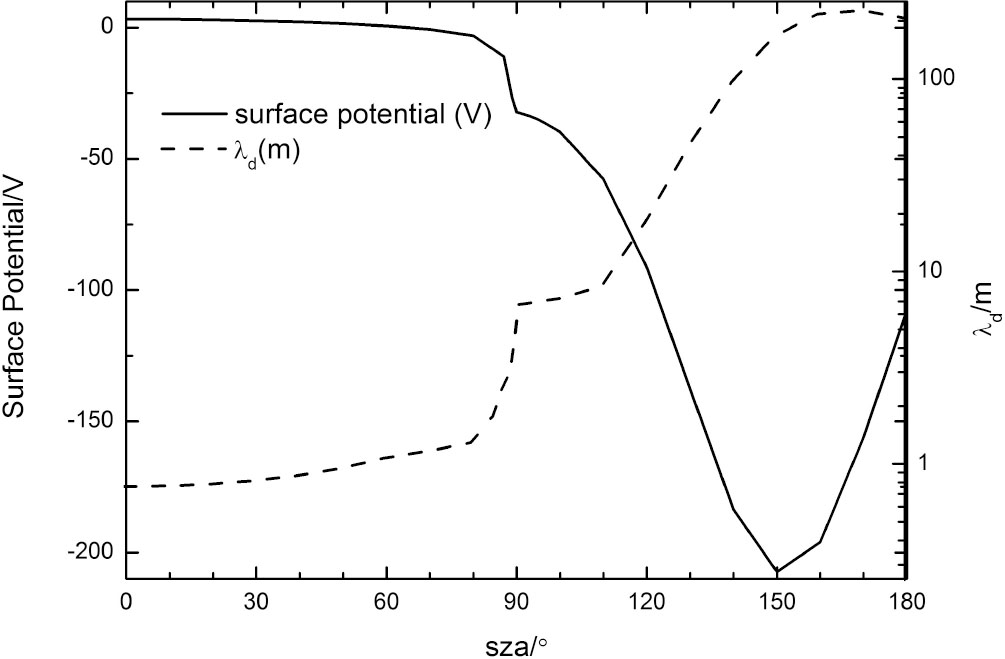
Fig. 5 Lunar surface potential and Debye length versus sza .
The changes in the surface potential and sheath thickness vs. sza imply that there is a horizontal gradient of the surface potential besides the vertical gradient. Theoretically, determining the 3D potential distribution needs a 3D plasma sheath model. However, a 3D global lunar surface sheath model will encounter difficulty because of the large difference between the vertical and horizontal scales. As a measure of the vertical scale, the Debye length is on the order of 100m (Fig. 5 ), corresponding to a vertical potential gradient of several V m −1 . The horizontal scale is measured in sza . For example, a 1° change in sza at the equator corresponds to a horizontal distance of 30 km. From Figure 5 , when sza changes from 0° to 80°, the surface potential has a change of ∼10 V, or the horizontal gradient is on the order of μV m −1 . Even at the terminator and on the nightside, the horizontal potential gradient is several mV/m, still 3 orders lower than the vertical gradient. So when solving a 3D global sheath model, if the computation domain is meshed according to the vertical scale, the horizontal gradient might be overwhelmed by numerical error. In other words, the horizontal gradient can be ignored compared with the vertical gradient, or the 3D model degrades into a 1D model. So, we can use the 1D model above to calculate the sheath potential profile at different sza to establish a 2D data set V ( x , sza ), from which the 3D electric field on the surface and in the sheath can be calculated globally.
In a spherical coordinate system ( r , θ , ϕ ) centered at the Moon's center, on the surface, θ = 0° is the zenith, and θ = 90°, ϕ = 0° correspond to the subsolar point. sza is a function of θ and ϕ , or sza = cos −1 (sin θ cos ϕ ). The electric field components can be calculated by differentiating the potential V ( x , sza ) according to Equation (5):
The results are shown in Figure 6 , with 6(a) and 6(b) showing the vertical and horizontal components respectively. From the figure, the vertical component is mostly positive on the dayside, pointing away from the surface. Near the terminator, the vertical component switches polarity, staying negative on the nightside. At the subpolar point, although the surface potential is not high, the vertical component is largest since the sheath is thinnest there. The horizontal component is actually the intensity, which stays positive as shown in Figure 6(b) . The distribution of the horizontal component is quite different from that of the vertical one. On the dayside, the horizontal component is usually very small, except around the terminator, where the horizontal component becomes significant. On the nightside, the horizontal component is larger due to the relatively quick variation of the potential vs. sza . At sza = 150°, the horizontal component is zero since the potential has no gradient there (Fig. 5 ). The horizontal component is further decomposed into eastward and northward components as shown in Figure 6(c) and Figure 6(d) respectively. The eastward component is in the magnitude of mV m −1 from the terminator to the nightside, pointing to midnight at sza < 150° and pointing to dawn/dusk at sza > 150°. The northward component is relatively large on the nightside (Fig. 6(d) ), at sza < 150°, pointing to the equator; at sza > 150°, pointing to the poles.
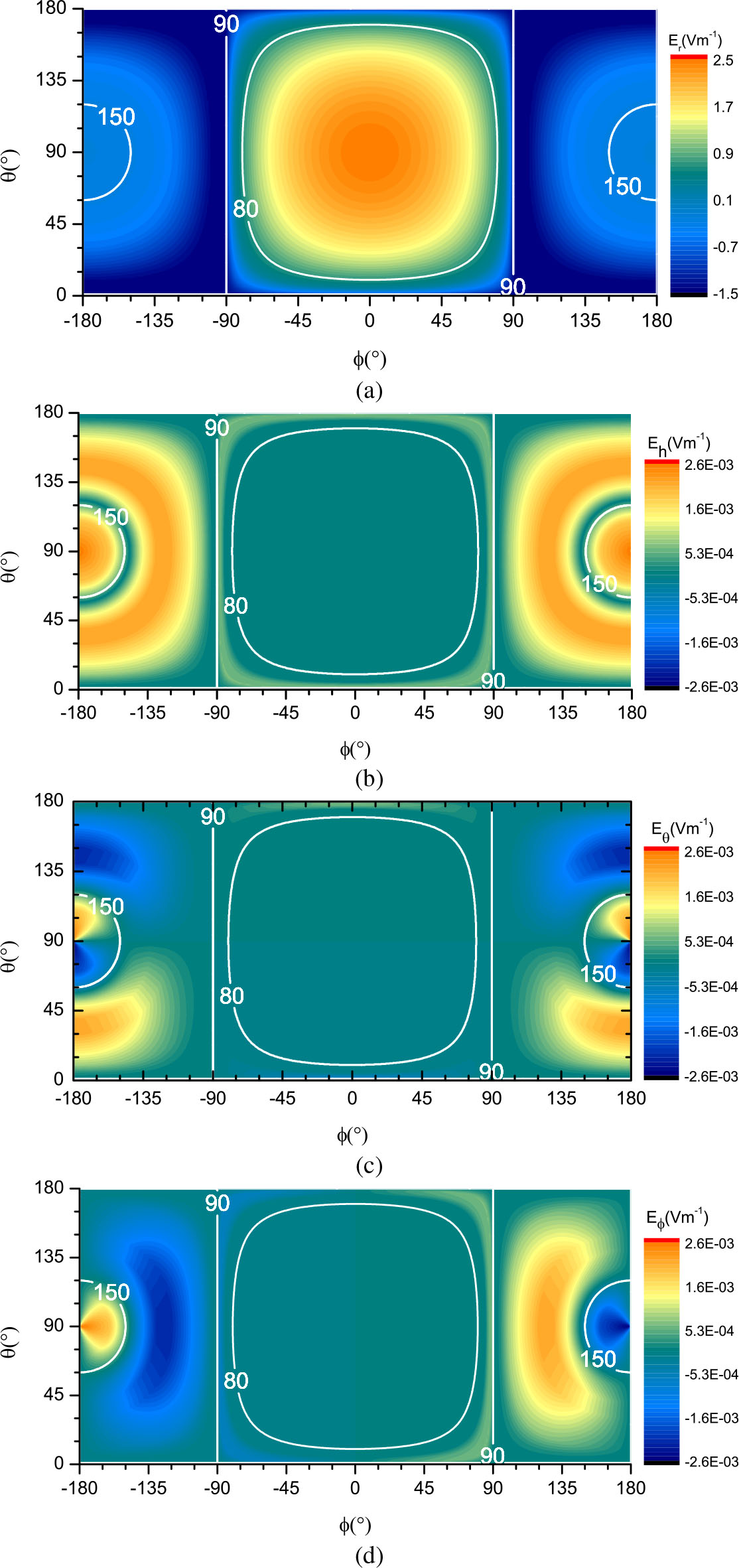
Fig. 6 Electric field components at the lunar surface: (a) vertical; (b) horizontal; (c) eastward; (d) northward. White lines show where sza = 80°, 90° and 150°. Here θ = 0° is the zenith; and θ = 90°, ϕ = 0° correspond to the subsolar point.
To check the details in Figure 6 , Figure 7 gives the vertical and horizontal components vs. sza around the terminator. From the dayside to the nightside, the vertical component switches from positive to negative at about 86°; while the horizontal component increases towards the terminator, decreases at sza ≥ 90° corresponding to the potential distribution in Figure 5 and increases again when the solar wind electron temperature increases deep into the wake. We should note here that the vertical and horizontal components are on different scales. Except in a very narrow region where the vertical component switches polarity, the magnitude of the vertical component is always much larger than that of the horizontal component.
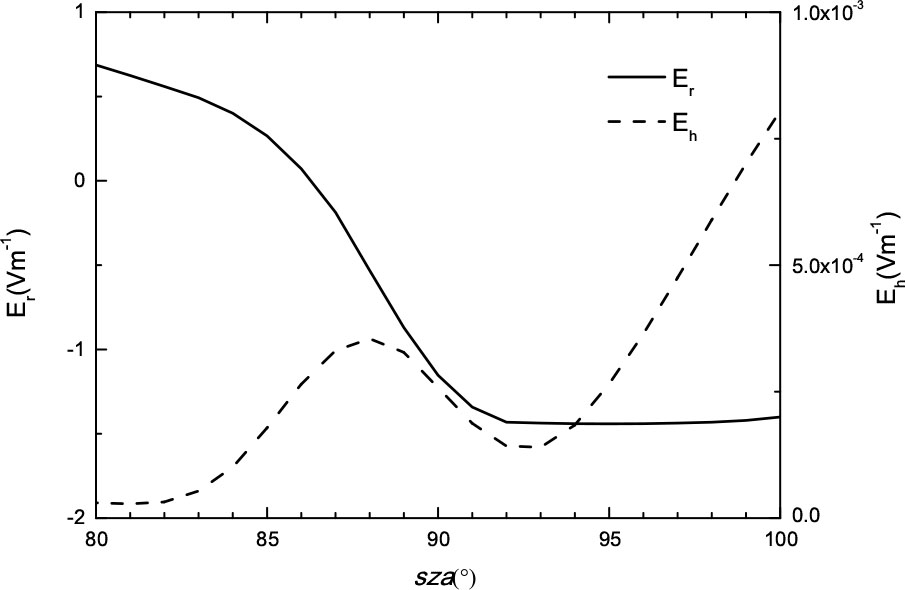
Fig. 7 The change of vertical and horizontal electric field components versus sza at the lunar surface around the terminator.
4. Conclusions and discussion
Based on the plasma sheath model, the lunar sheath is discussed at different solar zenith angles with different solar radiation and solar wind conditions. A global large scale electric field is deduced from the sheath profiles:
- 1) On the dayside, mostly the lunar surface sheath is dominated by photoelectrons emitted from the lunar surface. The surface potential is positive, and is modulated by the solar wind parameters. On the nightside, the sheath profile is controlled by the plasma distribution inside the lunar wake and the surface potential is negative. The surface sheath thickness varies with sza . At the subsolar point, the scale length of the sheath is smallest, while the scale length of the sheath on the nightside can be 100 times larger than that at the subsolar point.
- 2) The variations of sheath potential and thickness with sza result in the horizontal component of the electric field, besides the vertical one. On the dayside, the vertical component is the main component. The largest vertical component appears at the subsolar point with a magnitude of 1 V m −1 . The horizontal component enhances at the terminator and on the nightside, with a magnitude of mV m −1 . Around the terminator, the horizontal component points toward the nightside; while on the nightside, the horizontal field may point to or be away from midnight.
The potential distribution in our paper agrees with the Apollo observations (Freeman & Ibrahim 1975 ), and is also on the same order of magnitude as the PIC simulation results at the subsolar point (Poppe & Horányi 2010 ). However due to the lack of direct measurements of the surface electric field, detailed comparison has to be made in the future.
Throughout this paper, the effects of themagnetic field on the sheath and electric field are not considered. In the solar wind magnetic field of 10 nT, even a low energy lunar photoelectron has a gyroradius of about 300m, which is much larger than the sheath scale length on the dayside. On the nightside, sza = 120° for example, the gyroradius of the solar wind electron is about 1800m, which is also much larger than the sheath scale length of 20m. A charged particle moves almost along a straight line inside the sheath, hence can be taken as unmagnetized. A sheath model omitting the ambient magnetic field should be reasonable at the lunar surface if the lunar crustal fields are weak. However, in the strong lunar crustal field regions, the sheath might be magnetized. Through solar wind interaction with the crustal fields, the incoming plasma is also quite different from the undisturbed solar wind (Xie et al. 2015 ), and higher surface potential is expected (e.g., Saito et al. 2012 ). So, our results are not applicable to the strong crustal field regions.
The nightside sheath and electric field are determined by the plasma distribution in the lunar wake, especially by the electron temperature. According to Halekas et al. ( 2005 ), the various electron parameters are smoothly varying and nearly perfectly rotationally symmetric about the wake axis, but the electron temperature has a high level of variability in the central wake. In our calculation, the electron temperature in the region quasi-perpendicular to the magnetic field is adopted and smoothed as input. Considering the variability in plasma conditions in the central wake, uncertainty may arise, which should be further studied and confirmed by future observations.
To simplify the calculation, we have set the incoming plasma potential V 0 to zero in our work. Actually V 0 may drop to −300V in the central wake, and change with the solar wind parameters (Halekas et al. 2005 ). If V 0 has some non-zero value, the 1D sheath potential profile will have zero-offset, and an extra horizontal field will superpose on the present horizontal component according to the gradient in V 0 , which is almost zero on the dayside, but on the order of ∼0.2 mV m −1 deep in the wake.
Acknowledgements
This work is supported by the Key Research Program of the Chinese Academy of Sciences (Grant No. XDPB11).
A contributed paper from the International Symposium on Lunar and Planetary Science (ISLPS) on 2018 June 12–15 at Macau University of Science and Technology.
- Current Article
Purdue researchers experiment with harvesting static electricity as an energy source
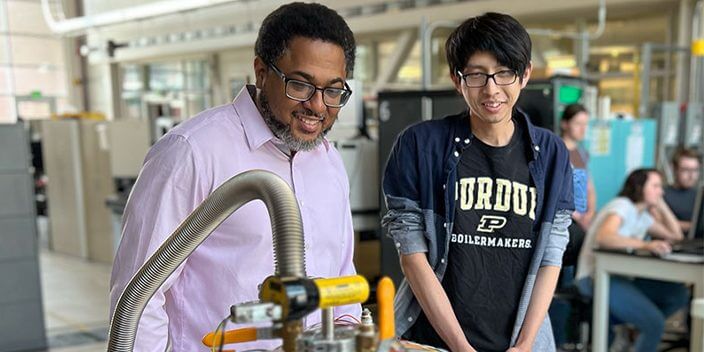
WEST LAFAYETTE, Ind. — Researchers in Purdue’s College of Engineering have developed an apparatus to generate and measure gas breakdown during contact electrification — the process responsible for static electricity. Their findings, published in Nature Communications , offer insight into the potential and limitations of this type of electricity when harvested as an energy source for a variety of devices, including e-textiles, wearables and smart packaging.
The apparatus, which is housed in an acrylic vacuum chamber, can isolate the potential materials used to make these devices and test their charge transfer capabilities without the use of an external voltage source. This creates the potential to improve the performance of these devices, as the apparatus measures the voltage generated between different pairs of materials.
The apparatus was designed and 3D printed by postdoctoral researcher Hongcheng Tao and associate professor of mechanical engineering James Gibert using additive manufacturing equipment at Ray W. Herrick Laboratories at Purdue University. Gibert has spent much of his career developing novel ways to generate electricity in unique situations. He has experimented with carboard boxes that power their own internal sensors, multifunction composite materials, and a tractor-trailer that harvests electricity from its own vibrations .
Tao and Gibert currently study the mechanisms behind triboelectric devices, which convert mechanical energy into electricity by rubbing two surfaces together. The surfaces can be either conductors or insulators, and they must be capable of reaching a high enough voltage to generate a sufficient electric charge without being damaged.
Triboelectric devices are still relatively in their infancy, with a number of researchers looking to further explore their potential. According to Gibert, there’s still a lot to learn about the physics behind triboelectrics.
“Typically, before you design one of these devices, you have to understand the application, including materials that are suitable for use and the power ranges required,” Gibert said. “So, our job is to investigate what can be achieved and what pairs of materials will give you the best voltage and subsequent current flow through a device.”
Quantifying that electric current between these materials can be difficult because, unlike similar apparatuses that use electrodes to demonstrate charge transfer, there is no external source generating a consistent, known voltage. So, Tao and Gibert had to find a workaround with their device that allowed them to calculate the voltage that is generated as the two materials make contact.
Their test apparatus consists of a small loading frame with motors that press and rub two sample materials together. The vacuum chamber removes all the air surrounding the device, and a single gas, such as nitrogen, is pumped into the chamber. As the samples make contact, they build up opposing charges. Then, when the samples are separated, the gas in the space between them breaks down and generates tiny sparks.
Tao and Gibert’s device measures that gas breakdown, or charge transfer, using a sensor that detects the Coulomb force — the attraction between the two charged objects. This measurement allows them to calculate the corresponding surface charge density that creates the attraction. From there, they can measure the charge transfer between the two samples.
The principle that governs this phenomenon, Paschen’s law, has been experimentally validated, but only between conductors. Tao and Gibert built their test apparatus to conduct the same experiment but with insulators.
“We have been assuming that Paschen’s law is valid when modeling gas breakdown in contact electrification, but there actually have not been sufficient experimental results to prove this,” Tao said. “So, we 3D printed every component to reconstruct an apparatus that was first implemented 30 years ago. All of the parts are flexible and customizable so we can ensure that all the samples align and the entire surface area is charged by contact.”
So far, Tao and Gibert have tested two combinations of materials: silicone-acrylic and copper-nylon, with the silicone-acrylic showing particular promise in generating a sufficient charge. When they perform these tests, they vary the pressure when the two samples make contact and the gap between them once they are separated. As a result of this process, they discovered some interesting phenomena.
“We found some regions where Paschen’s law didn’t hold,” Gibert said. “There are some regions where you have these discharges that can’t be explained by Paschen’s law. That’s an interesting finding that we’re hoping to look at in the future.”
So, while contributing to the development of triboelectric devices is the main objective for this research, Tao and Gibert are also excited about the basic science behind it all.
“The design of the device is largely an engineering challenge, but what’s really exciting is how the outcomes contribute to fundamental scientific knowledge. That’s the aspect we like most about this project,” Tao said. “I think it is very encouraging that a team of two people from an engineering department can still contribute to fundamental science research with a comparatively limited budget.”
This research was funded by an award from the National Science Foundation Division of Civil, Mechanical and Manufacturing Innovation (CMMI). CMMI funds innovative research that advances technologies related to manufacturing, materials and infrastructure.
About Purdue University Purdue University is a public research institution demonstrating excellence at scale. Ranked among top 10 public universities and with two colleges in the top four in the United States, Purdue discovers and disseminates knowledge with a quality and at a scale second to none. More than 105,000 students study at Purdue across modalities and locations, including nearly 50,000 in person on the West Lafayette campus. Committed to affordability and accessibility, Purdue’s main campus has frozen tuition 13 years in a row. See how Purdue never stops in the persistent pursuit of the next giant leap — including its first comprehensive urban campus in Indianapolis, the new Mitchell E. Daniels, Jr. School of Business, and Purdue Computes — at https://www.purdue.edu/president/strategic-initiatives .
Writer/Media contact: Lindsey Macdonald, [email protected]
Sources: James Gibert, [email protected]
Hongcheng Tao, [email protected]
Academia.edu no longer supports Internet Explorer.
To browse Academia.edu and the wider internet faster and more securely, please take a few seconds to upgrade your browser .
- We're Hiring!
- Help Center
Electric Potential
- Most Cited Papers
- Most Downloaded Papers
- Newest Papers
- Save to Library
- Last »
- Cellular mechanotransduction Follow Following
- ATP synthase Follow Following
- Electric Current Follow Following
- Receiving Operating Characteristic Follow Following
- Bioenergetics medicine, Bioenergetics medicine and immune system, Bioenergetics or quantum medicine Follow Following
- Lenz's Law Follow Following
- Electromotive Force Follow Following
- Energy Sources Follow Following
- Sonoporation Follow Following
- Electric Power Follow Following
Enter the email address you signed up with and we'll email you a reset link.
- Academia.edu Publishing
- We're Hiring!
- Help Center
- Find new research papers in:
- Health Sciences
- Earth Sciences
- Cognitive Science
- Mathematics
- Computer Science
- Academia ©2024
Electric Potential Response Characteristics of Coal Under Stress Wave Loading
- Original Paper
- Published: 15 March 2024
Cite this article
- Zesheng Zang 1 , 2 , 3 ,
- Zhonghui Li 1 , 2 , 3 ,
- Xin Zhang 1 , 2 , 3 ,
- Xiangguo Kong 4 ,
- Zhoujie Gu 1 , 2 , 3 ,
- Shan Yin 1 , 2 , 3 &
- Yue Niu 5 , 6
115 Accesses
Explore all metrics
Monitoring the electric potential (EP) response induced by coal deformation and rupture is essential to the safety of underground engineering under extreme load and stress wave disturbance conditions. In this study, the split Hopkinson pressure bar and EP acquisition systems were utilized for EP testing of coal under stress waves. The EP response under stress waves was investigated, the changes in accumulated charge caused by each stage of stress wave loading were analyzed, and the mechanism of EP signal generation is discussed. The results show that under stress waves, coal produces a significant EP signal, and the EP fluctuations correspond well to each stage of the stress wave. The EP and stress wave signals have a strong linear relationship when the stress wave is rising. During the descent of the stress wave, the EP decreases exponentially with time, showing a gradual decrease in the rate of EP decrease. The cumulative charge growth at each stage shows a “stable-surging-stable” change trend. Dislocation charged movement causes local polarization within the coal. Crack propagation leads to charge separation and free charge generation. These findings explain the mechanism of EP signal creation caused by stress waves. A mathematical model of the link between charge density and dynamic load stress is derived, indicating a strong positive correlation between the two parameters. The results of this research help to improve the reflection of coal seam stability through the EP response.
This is a preview of subscription content, log in via an institution to check access.
Access this article
Price includes VAT (Russian Federation)
Instant access to the full article PDF.
Rent this article via DeepDyve
Institutional subscriptions
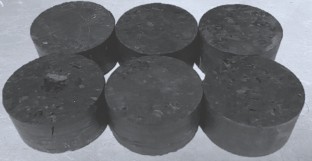
Similar content being viewed by others
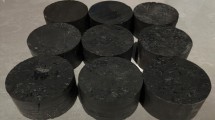
Electric Potential Response Characteristics and Constitutive Model of Coal Under Axial Static Load–Dynamic Load Coupling
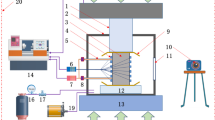
Study on electric potential response’s spatial distribution characteristics to damaging localization evolution of gas-bearing coal under load

Experimental study on pressure stimulated current response characteristics during damage evolution of water-bearing coal samples
Anastasiadis, C., Triantis, D., & Hogarth, C. A. (2007). Comments on the phenomena underlying pressure stimulated currents in dielectric rock materials. Journal of Materials Science, 42 (8), 2538–2542.
Article ADS CAS Google Scholar
Bruno, F., & Martillier, F. (2000). Test of high-resolution seismic reflection and other geophysical techniques on the Boup landslide in the Swiss Alps. Surveys in Geophysics, 21 (4), 333–348.
Article ADS Google Scholar
Carpinteri, A., & Borla, O. (2019). Acoustic, electromagnetic, and neutron emissions as seismic precursors: The lunar periodicity of low-magnitude seismic swarms. Engineering Fracture Mechanics, 210 , 29–41.
Article Google Scholar
Cartwright-Taylor, A., Vallianatos, F., & Sammonds, P. (2014). Superstatistical view of stress-induced electric current fluctuations in rocks. Physica A-Statistical Mechanics and Its Applications, 414 , 368–377.
Ding, Z., Feng, X. J., Wang, E. Y., Sa, L. B., Wang, D. M., Zhang, Q. M., et al. (2023a). Fracture response and damage evolution features of coal considering the effect of creep damage under dynamic loading. Engineering Failure Analysis, 148 , 107204.
Ding, Z., Feng, X. J., Wang, E. Y., Wei, Q. L., Zhao, X., & Hu, Q. J. (2023b). Acoustic emission response and evolution of precracked coal in the meta-instability stage under graded loading. Engineering Geology, 312 , 106930.
Fasani, G. B., Bozzano, F., Cardarelli, E., & Cercato, M. (2013). Underground cavity investigation within the city of Rome (Italy): A multi-disciplinary approach combining geological and geophysical data. Engineering Geology, 152 (1), 109–121.
Feng, J. J., Wang, E. Y., Huang, Q. S., Ding, H. C., & Zhang, X. Y. (2020). Experimental and numerical study of failure behavior and mechanism of coal under dynamic compressive loads. International Journal of Mining Science and Technology, 30 (5), 613–621.
Freund, F. (2011). Pre-earthquake signals: Underlying physical processes. Journal of Asian Earth Sciences, 41 (4–5), 383–400.
Griffiths, L., Lengline, O., Heap, M. J., Baud, P., & Schmittbuhl, J. (2018). Thermal cracking in westerly granite monitored using direct wave velocity, coda wave interferometry, and acoustic emissions. Journal of Geophysical Research-Solid Earth, 123 (3), 2246–2261.
Gu, Z. J., Shen, R. X., Liu, Z. T., Zhao, E. L., Chen, H. L., Yuan, Z. C., et al. (2023a). Dynamic characteristics of coal under triaxial constraints based on the split–hopkinson pressure bar test system. Natural Resources Research, 32 (2), 587–601.
Gu, Z. J., Shen, R. X., Liu, Z. T., Zhou, X., Li, X. L., Zang, Z. S., et al. (2023b). Strain rate effect and mechanical constitutive model of coal samples under dynamic load. Natural Resources Research, 32 , 2769–2785.
Guo, Z. Q. (1988). Electron emission during rock fracture. Chinese Journal of Geophysics, 31 (05), 566–571.
Google Scholar
Guven, C., Wolf, L. W., Tuttle, M. P., & Rogers, S. R. (2023). The influence of sedimentary architecture on the formation of earthquake-induced liquefaction features: A case study in the New Madrid seismic zone. Engineering Geology, 312 , 106946.
Ida, Y. (1972). Cohesive force across the tip of a longitudinal-shear crack and Griffith’s specific surface energy. Journal of Geophysical Research (1896–1977), 77 (20), 3796–3805.
Kong, X. G., Zhan, M. Z., Cai, Y. C., Ji, P. F., He, D., Zhao, T. S., et al. (2023a). Precursor signal identification and acoustic emission characteristics of coal fracture process subjected to uniaxial loading. Sustainability, 15 (15), 11581.
Kong, X. G., Zhan, M. Z., Cai, Y. C., Zhang, C. L., Wang, E. Y., Li, S. G., et al. (2023b). Experimental and simulation researches of loaded stress and gas environment on dynamics properties of gas-bearing coal during impact failure process. Bulletin of Engineering Geology and the Environment, 83 (1), 16.
Kyriazopoulos, A., Anastasiadis, C., Triantis, D., & Brown, C. J. (2011). Non-destructive evaluation of cement-based materials from pressure-stimulated electrical emission—Preliminary results. Construction and Building Materials, 25 (4), 1980–1990.
Kyriazopoulos, A., Stavrakas, I., Anastasiadis, C., & Triantis, D. (2005). Pressure stimulated current (PSC) recordings on cement mortar and marble. In Biolek, D., & Mastorakis, N. (Eds.), Proceedings of the 4th WSEAS International Conference On Applications Of Electrical Engineering (pp. 12–15). Athens: World Scientific and Engineering Acad and Soc. Retrieved 10 October, 2022, from https://www.webofscience.com/wos/alldb/full-record/WOS:000230049300003 .
Li, D. Y., Han, Z. Y., Sun, X. L., Zhou, T., & Li, X. B. (2019a). Dynamic mechanical properties and fracturing behavior of marble specimens containing single and double flaws in SHPB tests. Rock Mechanics and Rock Engineering, 52 (6), 1623–1643.
Li, D. Y., Han, Z. Y., Zhu, Q. Q., Zhang, Y., & Ranjith, P. G. (2019b). Stress wave propagation and dynamic behavior of red sandstone with single bonded planar joint at various angles. International Journal of Rock Mechanics and Mining Sciences, 117 , 162–170
Li, D. X., Wang, E. Y., Ju, Y. Q., & Wang, D. M. (2021a). Laboratory investigations of a new method using pressure stimulated currents to monitor concentrated stress variations in coal. Natural Resources Research, 30 (1), 707–724.
Article CAS Google Scholar
Li, D. X., Wang, E. Y., Kong, X. G., Zhao, S., Kong, Y. H., Wang, X. R., et al. (2018). Mechanical properties and electromagnetic radiation characteristics of concrete specimens after exposed to elevated temperatures. Construction and Building Materials, 188 , 381–390.
Li, D. X., Wang, E. Y., Li, Z. H., Ju, Y. Q., Wang, D. M., & Wang, X. Y. (2021b). Experimental investigations of pressure stimulated currents from stressed sandstone used as precursors to rock fracture. International Journal of Rock Mechanics and Mining Sciences, 145 , 104841.
Li, H. R., Shen, R. X., Wang, E. Y., Li, D. X., Li, T. X., Chen, T. Q., & Hou, Z. H. (2020a). Effect of water on the time-frequency characteristics of electromagnetic radiation during sandstone deformation and fracturing. Engineering Geology, 265 , 105451.
Li, M., Wang, H. T., Wang, D. M., & Shao, Z. L. (2020b). Experimental study on characteristics of surface potential and current induced by stress on coal mine sandstone roof. Engineering Geology, 266 , 105468.
Li, Q. M., & Meng, H. (2003). About the dynamic strength enhancement of concrete-like materials in a split Hopkinson pressure bar test. International Journal of Solids and Structures, 40 (2), 343–360.
Article MathSciNet Google Scholar
Li, X. L., Li, Z. H., Yin, S., Lei, Y. Y., Niu, Y., Tian, H., et al. (2023a). Experimental study on infrared thermal response characteristics of water-bearing concrete under drop hammer impact. Infrared Physics & Technology, 135 , 104899.
Li, X. L., Liu, Z. T., Feng, X. J., Zhang, H. J., & Feng, J. J. (2021c). Effects of acid sulfate and chloride ion on the pore structure and mechanical properties of sandstone under dynamic loading. Rock Mechanics and Rock Engineering, 54 (12), 6105–6121.
Li, X. L., Liu, Z. T., Zhao, E. L., Liu, Y. B., Feng, X. J., & Gu, Z. J. (2023b). Experimental study on the damage evolution behavior of coal under dynamic Brazilian splitting tests based on the Split Hopkinson pressure bar and the digital image correlation. Natural Resources Research, 32 (3), 1435–1457.
Li, X. L., Zhao, E. L., Liu, Z. T., Liu, Y. B., Feng, X. J., & Gu, Z. J. (2023c). Experimental study on multiple propagation characteristics of stress wave and surface displacement behavior in coal based on SHPB and DIC. Bulletin of Engineering Geology and the Environment, 82 (7), 246.
Li, Z. H. (2010). Study on Surface potential effect and its mechanism of coal during deformation and fracture under load. Journal of China University of Mining & Technology, 39 (1), 153–154.
Li, Z. H., Wang, E. Y., Liu, Z. T., Song, X. Y., & Li, Y. N. (2009). Study on characteristics and rules of surface potential during coal fracture. Journal of China University of Mining & Technology, 38 (02), 187–192.
CAS Google Scholar
Lv, X. F., Pan, Y. S., Xiao, X. C., & Wang, A. W. (2013). Barrier formation of micro-crack interface and piezoelectric effect in coal and rock masses. International Journal of Rock Mechanics and Mining Sciences, 64 , 1–5.
Ma, L. J., Wu, J. W., Wang, M. J., Dong, L., & Wei, H. Z. (2020). Dynamic compressive properties of dry and saturated coral rocks at high strain rates. Engineering Geology, 272 , 105615.
Niu, Y., Li, Z. H., Wang, E. Y., Shen, R. S., Cheng, Z. H., Gao, X. Y., et al. (2020). Study on characteristics of EP responsing to coal mining. Engineering Fracture Mechanics, 224 , 106780.
Niu, Y., Wang, C. J., Wang, E. Y., & Li, Z. H. (2019). Experimental study on the damage evolution of gas-bearing coal and its electric potential response. Rock Mechanics and Rock Engineering, 52 (11), 4589–4604.
Niu, Y., Wang, E. Y., Li, Z. H., Gao, F., Zhang, Z. Z., Li, B. L., & Zhang, X. (2022). Identification of coal and gas outburst-hazardous zones by electric potential inversion during mining process in deep coal seam. Rock Mechanics and Rock Engineering, 55 (6), 3439–3450.
Ren, H. X., Chen, X. F., & Huang, Q. H. (2012). Numerical simulation of coseismic electromagnetic fields associated with seismic waves due to finite faulting in porous media. Geophysical Journal International, 188 (3), 925–944.
Revil, A., Naudet, V., & Meunier, J. D. (2004). The hydroelectric problem of porous rocks: Inversion of the position of the water table from self-potential data. Geophysical Journal International, 159 (2), 435–444.
Scoville, J., Sornette, J., & Freund, F. T. (2015). Paradox of peroxy defects and positive holes in rocks Part II: Outflow of electric currents from stressed rocks. Journal of Asian Earth Sciences, 114 , 338–351.
Slifkin, L. (1993). Seismic electric signals from displacement of charged dislocations. Tectonophysics, 224 , 149–152.
Stavrakas, I., Triantis, D., Agioutantis, Z., Maurigiannakis, S., Saltas, V., Vallianatos, F., & Clarke, M. (2004). Pressure stimulated currents in rocks and their correlation with mechanical properties. Natural Hazards and Earth System Sciences, 4 (4), 563–567.
Stergiopoulos, C., Stavrakas, I., Hloupis, G., Triantis, D., & Vallianatos, F. (2013). Electrical and acoustic emissions in cement mortar beams subjected to mechanical loading up to fracture. Engineering Failure Analysis, 35 , 454–461.
Sutula, D., Kerfriden, P., van Dam, T., & Bordas, S. P. A. (2018). Minimum energy multiple crack propagation. Part I: Theory and state of the art review. Engineering Fracture Mechanics, 191 , 205–224.
Taylor, D., Cornetti, P., & Pugno, N. (2005). The fracture mechanics of finite crack extension. Engineering Fracture Mechanics, 72 (7), 1021–1038.
Tian, H., Li, Z. H., Shen, X. F., Zang, Z. S., Song, J. J., & Zhang, Q. C. (2021). Identification method of infrared radiation precursor information of coal sample failure and instability under uniaxial compression. Infrared Physics & Technology, 119 , 103957.
Tresoldi, G., Arosio, D., Hojat, A., Longoni, L., Papini, M., & Zanzi, L. (2019). Long-term hydrogeophysical monitoring of the internal conditions of river levees. Engineering Geology, 259 , 105139.
Triantis, D., Pasiou, E. D., Stavrakas, I., Dakanali, I., & Kourkoulis, S. K. (2017). Correlation of pressure stimulated currents and acoustic emissions during 3PB of cement-mortar beams and the role of loading rate. In Iacoviello, F., Susmel, L., Firrao, D., & Ferro, G. (Eds.), Xxiv Italian group of fracture conference, 2017 (Vol. 3, pp. 346–353). Amsterdam: Elsevier Science Bv. https://doi.org/10.1016/j.prostr.2017.04.027 .
Triantis, D., Stavrakas, I., Anastasiadis, C., Kyriazopoulos, A., & Vallianatos, F. (2006). An analysis of pressure stimulated currents (PSC), in marble samples under mechanical stress. Physics and Chemistry of the Earth, 31 (4–9), 234–239.
Uyeda, S., Nagao, T., & Kamogawa, M. (2009). Short-term earthquake prediction: Current status of seismo-electromagnetics. Tectonophysics, 470 (3–4), 205–213.
Vallianatos, F., Triantis, D., Tzanis, A., Anastasiadis, C., & Stavrakas, A. (2004). Electric earthquake precursors: From laboratory results to field observations. Physics and Chemistry of the Earth, 29 (4–9), 339–351.
Wang, E., He, X., Wei, J., Nie, B., & Song, D. (2011). Electromagnetic emission graded warning model and its applications against coal rock dynamic collapses. International Journal of Rock Mechanics and Mining Sciences, 48 (4), 556–564.
Wang, P., Xu, J. Y., Liu, S., Wang, H. Y., & Liu, S. H. (2016). Static and dynamic mechanical properties of sedimentary rock after freeze-thaw or thermal shock weathering. Engineering Geology, 210 , 148–157.
Xie, H. P., Gao, M. Z., Zhang, R., Peng, G. Y., Wang, W. Y., & Li, A. Q. (2019). Study on the mechanical properties and mechanical response of coal mining at 1000m or deeper. Rock Mechanics and Rock Engineering, 52 (5), 1475–1490.
Yang, J. H., Lu, W. B., Chen, M., Yan, P., & Zhou, C. B. (2013). Microseism induced by transient release of in situ stress during deep rock mass excavation by blasting. Rock Mechanics and Rock Engineering, 46 (4), 859–875.
Yao, Q. L., Tang, C. J., Xia, Z., Liu, X. L., Zhu, L., Chong, Z. H., & Hui, X. D. (2020). Mechanisms of failure in coal samples from underground water reservoir. Engineering Geology, 267 , 105494.
Yfantis, G., Pytharouli, S., Lunn, R. J., & Carvajal, H. E. M. (2021). Microseismic monitoring illuminates phases of slope failure in soft soils. Engineering Geology, 280 , 105940.
Yin, S., Li, Z. H., Song, D. Z., He, X. Q., Qiu, L. M., Lou, Q., & Tian, H. (2021). Experimental study on the infrared precursor characteristics of gas-bearing coal failure under loading. International Journal of Mining Science and Technology, 31 (5), 901–912.
Zang, Z. S., Li, Z. H., Niu, Y., Tian, H., Zhang, X., Li, X. L., & Ali, M. (2021). Energy dissipation and electromagnetic radiation response of sandstone samples with a pre-existing crack of various inclinations under an impact load. Minerals, 11 (12), 1363.
Zang, Z. S., Li, Z. H., Niu, Y., & Yin, S. (2024). Experimental investigation of the fracture and damage evolution characteristics of flawed coal based on electric potential and acoustic emission parameter analyses. Engineering Fracture Mechanics, 295 , 109740.
Zang, Z. S., Li, Z. H., Zhao, E. L., Kong, X. G., Niu, Y., & Yin, S. (2023). Electric potential response characteristics and constitutive model of coal under axial static load-dynamic load coupling. Natural Resources Research, 32 (6), 2821–2844.
Zhang, G. R., & Wang, E. Y. (2023). Risk identification for coal and gas outburst in underground coal mines: A critical review and future directions. Gas Science and Engineering, 118 , 205106.
Zhang, Q. B., & Zhao, J. (2014). A review of dynamic experimental techniques and mechanical behaviour of rock materials. Rock Mechanics and Rock Engineering, 47 (4), 1411–1478.
Zhang, X., Li, Z. H., Niu, Y., Cheng, F. Q., Ali, M., & Bacha, S. (2019). An experimental study on the precursory characteristics of EP before sandstone failure based on critical slowing down. Journal of Applied Geophysics, 170 , 103818.
Zhao, E. L., Wang, E. Y., & Chen, H. P. (2023). Study on dynamic parameters and energy dissipation characteristics of coal samples under dynamic load and temperature. Processes, 11 (12), 3326.
Zhou, X., Liu, X. F., Wang, X. R., Liu, Y. B., Xie, H., & Du, P. F. (2023a). Acoustic emission characteristics of coal failure under triaxial loading and unloading disturbance. Rock Mechanics and Rock Engineering, 56 (2), 1043–1061.
Zhou, X., Liu, X. F., Wang, X. R., Xie, H., & Du, P. F. (2023b). Failure characteristics and mechanism of coal under the coupling between different confining pressures and disturbance loading. Bulletin of Engineering Geology and the Environment, 82 (12), 442.
Zhou, Y. X., Xia, K., Li, X. B., Li, H. B., Ma, G. W., Zhao, J., et al. (2012). Suggested methods for determining the dynamic strength parameters and mode-I fracture toughness of rock materials. International Journal of Rock Mechanics and Mining Sciences, 49 , 105–112.
Download references
Acknowledgments
This research was supported by the National Key R&D Program of China (2022YFC3004705), the National Natural Science Foundation of China (52074280), the Key Projects of National Natural Science Foundation of China (51934007), the Development of Jiangsu Higher Education Institutions (PAPD), the Jiangsu Funding program for Excellent postdoctoral Talent (2022ZB505), the Postgraduate Research & Practice Innovation Program of Jiangsu Province (KYCX23_2858), the Graduate Innovation Program of China University of Mining and Technology (2023WLKXJ144), and the Fundamental Research Funds for the Central Universities (2023XSCX037). The authors express their gratitude to the editors and reviewers for their helpful comments, which helped to make this paper better.
Author information
Authors and affiliations.
National Engineering Research Center for Coal Gas Control, China University of Mining and Technology, Xuzhou, 221116, Jiangsu, China
Zesheng Zang, Zhonghui Li, Xin Zhang, Zhoujie Gu & Shan Yin
Key Laboratory of Gas and Fire Control for Coal Mines of Ministry of Education, China University of Mining and Technology, Xuzhou, 221116, Jiangsu, China
School of Safety Engineering, China University of Mining and Technology, Xuzhou, 221116, Jiangsu, China
College of Safety Science and Engineering, Xian University of Science and Technology, Xi’an, 710054, Shaanxi, China
Xiangguo Kong
State Key Laboratory for GeoMechanics and Deep Underground Engineering, China University of Mining and Technology, Xuzhou, 221116, China
Frontier Scientific Research Center of Fluidized Mining of Deep Resources, China University of Mining and Technology, Xuzhou, 221116, China
You can also search for this author in PubMed Google Scholar
Corresponding author
Correspondence to Zhonghui Li .
Ethics declarations
Conflict of interest.
The authors declared that there is no conflict of interest.
Rights and permissions
Springer Nature or its licensor (e.g. a society or other partner) holds exclusive rights to this article under a publishing agreement with the author(s) or other rightsholder(s); author self-archiving of the accepted manuscript version of this article is solely governed by the terms of such publishing agreement and applicable law.
Reprints and permissions
About this article
Zang, Z., Li, Z., Zhang, X. et al. Electric Potential Response Characteristics of Coal Under Stress Wave Loading. Nat Resour Res (2024). https://doi.org/10.1007/s11053-024-10324-6
Download citation
Received : 19 November 2023
Accepted : 27 January 2024
Published : 15 March 2024
DOI : https://doi.org/10.1007/s11053-024-10324-6
Share this article
Anyone you share the following link with will be able to read this content:
Sorry, a shareable link is not currently available for this article.
Provided by the Springer Nature SharedIt content-sharing initiative
- Split Hopkinson pressure bar
- Stress waves
- Electric potential response
- Electrification mechanism
- Mechanical behavior
- Find a journal
- Publish with us
- Track your research
Physical Review Research
- Collections
- Editorial Team
- Open Access
Transverse-electric surface plasmon polaritons in periodically modulated graphene
Zeeshan ahmad, sang soon oh, and egor a. muljarov, phys. rev. research 6 , 023185 – published 20 may 2024.
- No Citing Articles
- INTRODUCTION
- THEORETICAL MODEL
- CONCLUSIONS
- ACKNOWLEDGMENTS
Transverse-electric (TE) surface plasmon polaritons are unique eigenmodes of a homogeneous graphene layer that are tunable with the chemical potential and temperature. However, as their dispersion curve spectrally lies below the light line, they cannot be resonantly excited by an externally incident wave. Here, we propose a way of exciting the TE modes and tuning their peaks in the transmission by introducing a one-dimensional graphene grating. Using the scattering-matrix formalism, we show that periodic modulation of graphene makes transmission more pronounced, potentially allowing for experimental observation of the TE modes. Furthermore, we propose the use of turbostratic graphene to enhance the role of the surface plasmon polaritons in optical spectra.
- Received 8 February 2023
- Revised 22 January 2024
- Accepted 1 March 2024
DOI: https://doi.org/10.1103/PhysRevResearch.6.023185

Published by the American Physical Society under the terms of the Creative Commons Attribution 4.0 International license. Further distribution of this work must maintain attribution to the author(s) and the published article's title, journal citation, and DOI.
Published by the American Physical Society
Physics Subject Headings (PhySH)
- Research Areas
- Physical Systems
Authors & Affiliations
- School of Physics and Astronomy, Cardiff University, Cardiff CF24 3AA, United Kingdom
- * [email protected]
Article Text
Vol. 6, Iss. 2 — May - July 2024
Subject Areas
- Condensed Matter Physics
- Metamaterials
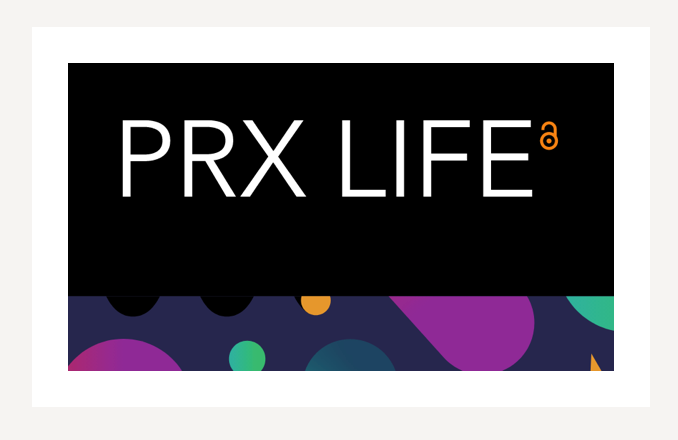
Authorization Required
Other options.
- Buy Article »
- Find an Institution with the Article »
Download & Share
Schematic of a graphene grating (a) in a perspective view and (b) in the x z -plane side view. An infinitesimally thin graphene layer at z = 0 is periodically modulated along the x axis but homogeneous along the y axis. Arrows labeled A n , B n , C n , and D n schematically represent incoming or outgoing coefficients of diffracted plane waves with an angle of incidence not necessarily normal.
Electronic dispersion, shown as electronic energy versus two-dimensional electronic momentum, of graphene near the K point with nonzero chemical potential μ and zero temperature. The darker colored region of surface of cone depicts energies occupied by charge carriers.
(a) Conductivity of graphene at zero temperature ( T = 0 ), sharing the frequency axis with dispersion in (b). The frequency of the interband dip in imaginary part of conductivity is affected by μ but not d . (b) Real part of the frequency of the TE mode dispersion of the homogeneous graphene structure folded into the first Brillouin zone, shown for G 1 = 2 , 2.3, and 2.6 (thin, medium and thick blue lines, respectively). The size of the first Brillouin zone, and consequently the frequency of mode crossing at Q = 0 , is controlled by μ d . The TE mode frequency is close to the light line due to small value of the fine-structure constant. (c) The period of grating, d , required for normalized frequency Ω = 2 , various chemical potentials μ , and corresponding frequencies ω (legend) as a function of desired incidence angle θ = arcsin ( q / ω ) in degrees, using Eq. ( 21 ).
Transmission spectra ( 1 − T 0 ) for zeroth diffraction order (close-to-normal incidence) when a TE-polarized light with parallel wave number Q is incident on the grating with b / d = 0.3 , Δ = 0 , for (a) single-layer graphene with σ / 2 π = σ intra + σ inter , (b) single-layer graphene without interband conductivity σ / 2 π = σ intra , and (c) turbostratically stacked graphene with σ / 2 π = N ( σ intra + σ inter ) , for N = 10 . The dashed red line indicates T 0 = 0 . Spectra for Q ≠ 0 are offset by powers of 10 in increasing order of Q . Red and black vertical dash-dotted lines indicate the real parts of the bright and dark TE SPP resonance frequencies for Q = 0 , which are solutions of Eqs. ( 34 ) and ( 33 ), respectively.
Approximation of transmission (red solid line) using Eq. ( 35 ) showing consistency with the TE peak for Q = 0 and Δ = 0 (blue solid line). Red and black dashed lines indicate the real part of the frequencies of the bright and dark TE SPP modes, obtained using Eq. ( 34 ) and Eq. ( 33 ), respectively. The frequency gap between the dashed lines is approximately 1.47 × 10 − 4 .
Transmission spectra ( 1 − T 0 ) for zeroth diffraction order when a TE-polarized light is normally incident ( Q = 0 ) on grating with b / d = 0.3 , for Δ = 0 , shown from zero temperature (red) to finite temperature (green), for (a) single-layer graphene and (b) turbostratically stacked graphene. Red and green dash-dotted lines indicate T 0 = 0 for zero temperature and T el = 0.3 K (single) or T el = 3 K (turbostratic), respectively.
Transmission spectra for single-layer graphene ( N = 1 ), for the [(a), (b)] minus-first diffraction order, [(c), (d)] zeroth diffraction order, and [(e), (f)] first diffraction order, for the period d adjusted so that Δ = − 1 ( G 1 = 1 ). Panels (b) and (d) zoom in the spectral features near Ω = G 1 in (a) and (c), respectively, for Q = 0 . (g) Absorption calculated using Eq. ( B1 ), with waterfall increment of 0.02 . A ( Ω < 2 ) = 0 for zero temperature. The gray solid lines correspond to the forbidden frequency range where Eq. ( 15 ) is not satisfied, and the curve has the meaning of the near-field amplitude.
As Fig. 7 but for Δ = − 2 + 1.667 ( G 1 = 1.667 ), illustrating attenuation of near-field and transmission amplitudes.
As Fig. 7 but for Δ = 0 ( G 1 = 2 ), illustrating the amplification of the near-field and transmission amplitudes.
Transmission spectra for turbostratic ( N = 10 ) graphene, for the [(a), (b)] minus-first diffraction order, [(c), (d)] zeroth diffraction order, and [(e), (f)] first diffraction order for detuning Δ = − 1 ( G 1 = 1 ). Panels (b) and (d) zoom in the spectral features near Ω = G 1 in (a) and (c), respectively, for Q = 0 . Similarly, panel (f) is a zoom in of panel (e) at Q close to but not zero. Gray regions and curves indicate forbidden frequency regions. (g) Absorption spectrum obtained using Eq. ( B1 ), with waterfall increment of 0.2 .
As Fig. 10 but for Δ = − 2 + 1.667 ( G 1 = 1.667 ).
As Fig. 10 but for Δ = 0 ( G 1 = 2 ).
As Fig. 10 but for Δ = 0.5 ( G 1 = 2.5 ).
Sign up to receive regular email alerts from Physical Review Research
Reuse & Permissions
It is not necessary to obtain permission to reuse this article or its components as it is available under the terms of the Creative Commons Attribution 4.0 International license. This license permits unrestricted use, distribution, and reproduction in any medium, provided attribution to the author(s) and the published article's title, journal citation, and DOI are maintained. Please note that some figures may have been included with permission from other third parties. It is your responsibility to obtain the proper permission from the rights holder directly for these figures.
- Forgot your username/password?
- Create an account
Article Lookup
Paste a citation or doi, enter a citation.
- International
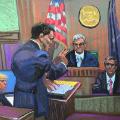
Trump's hush money trial
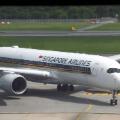
Singapore Airlines flight
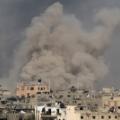
Middle East turmoil
The latest on the massive solar storm
By Angela Fritz, Elise Hammond and Chris Lau, CNN
Incredible lighthouse picture from Maine
From CNN's Chris Lau
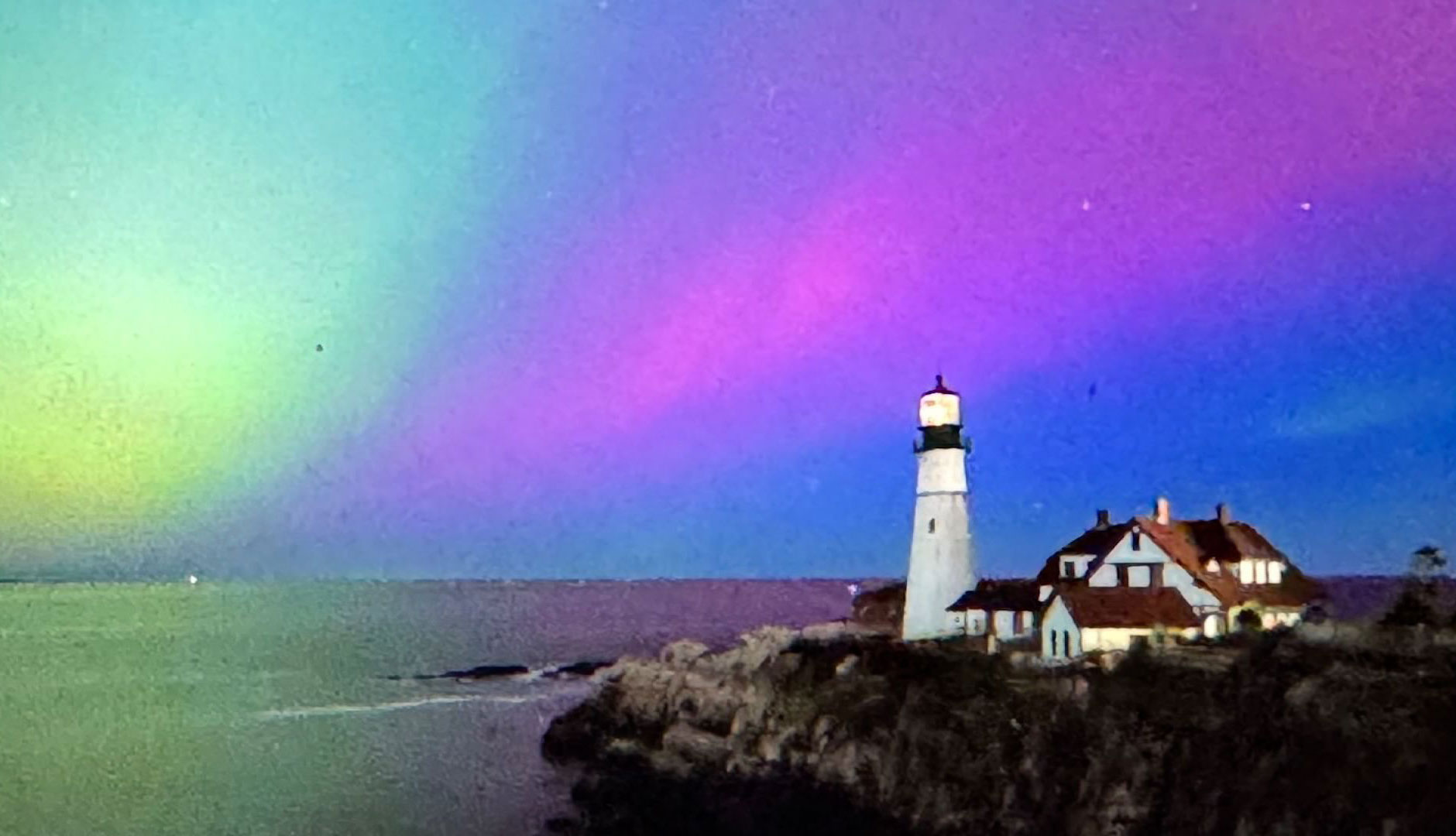
Among a flurry of surreal images capturing the dazzling auroras is one taken by Benjamin Williamson of a lighthouse in Portland, Maine.
"It's one of the most incredible things I've ever seen, the awe and wonder," Williamson told CNN.
He said he used a long-exposure technique to snap the shot, but did not edit it.
Watch the full interview with Williamson here .
Things could be about to ramp up
If you still haven't seen the aurora, hold on for another 30 minutes to an hour, according to CNN meteorologist Chad Myers.
The next wave of coronal mass ejections, or CMEs, which cause the aurora, is about to arrive, he said.
"Just wait a minute because things are going to start to ramp up here," he said, adding that the increase could arrive "anytime now." "When it comes, get outside, get ready, put your coat on."
For those who are too busy to witness the phenomenon tonight, Myers said the aurora is expected to last three nights.
Why does the aurora last for a weekend?
By CNN's Chris Lau
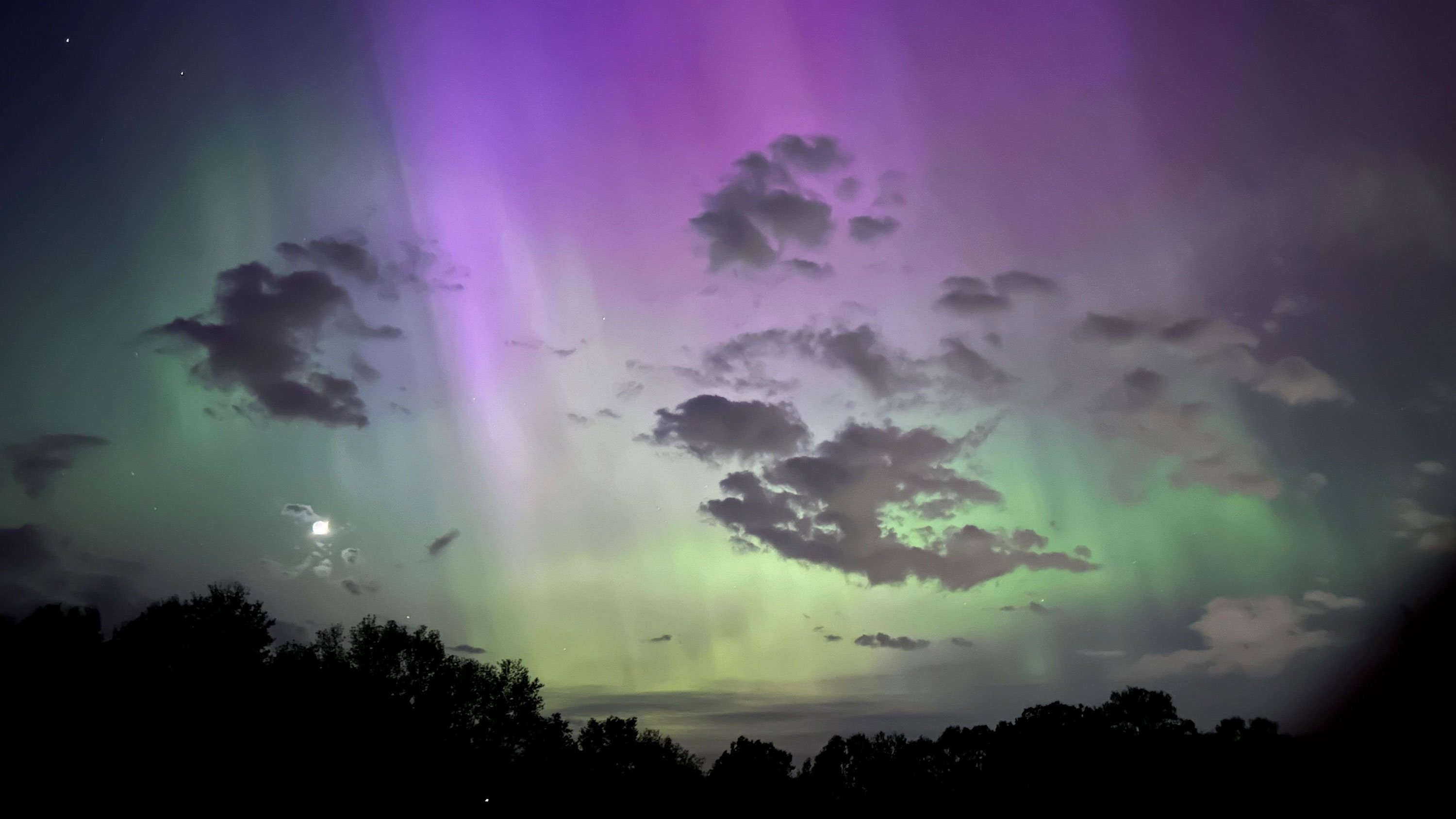
Generally, it takes just eight minutes for light to travel 93 million miles to the Earth from the sun, but astrophysicist Janna Levin said the energized particles causing the current wave of aurora travel a lot slower, causing the phenomenon to last for the weekend.
"Some of these mass ejections are trillions of kilograms," she said. "They're slower. So they're taking longer, but still hours, maybe tens of hours."
Here's how the solar storm looks in the South and on the East Coast
The aurora was visible across the East Coast and in the South Friday.
Here's how it looked in Chester, South Carolina.
Down in Florida, waves of color swam through the sky.
Up north in New Jersey, a purple-ish haze could be seen in the sky.
Will solar storms get more intense and risky in the future?
The answer is probably not in the short term, according to astrophysicist Hakeem Oluseyi.
He said scientists study what is constantly happening on the surface of the sun and have found a pattern.
“Geological data shows us that in the past the sun was way more active than it is today. It has cycles where it goes very quiet ... and you have events that show that the solar activity was much, much greater,” he told CNN. “So there's no evidence that we're going to see those big maxima this cycle."
But the astrophysicist also spoke of a caveat - the limitations of modern science.
“Even though it's predictable in the short term, we still don't quite understand what creates the magnetic fields in the sun,” he said, adding: “That's why NASA has so many satellites looking at the sun.”
In Pictures: Auroras light the sky during rare solar storm
From CNN Digital's Photo Team
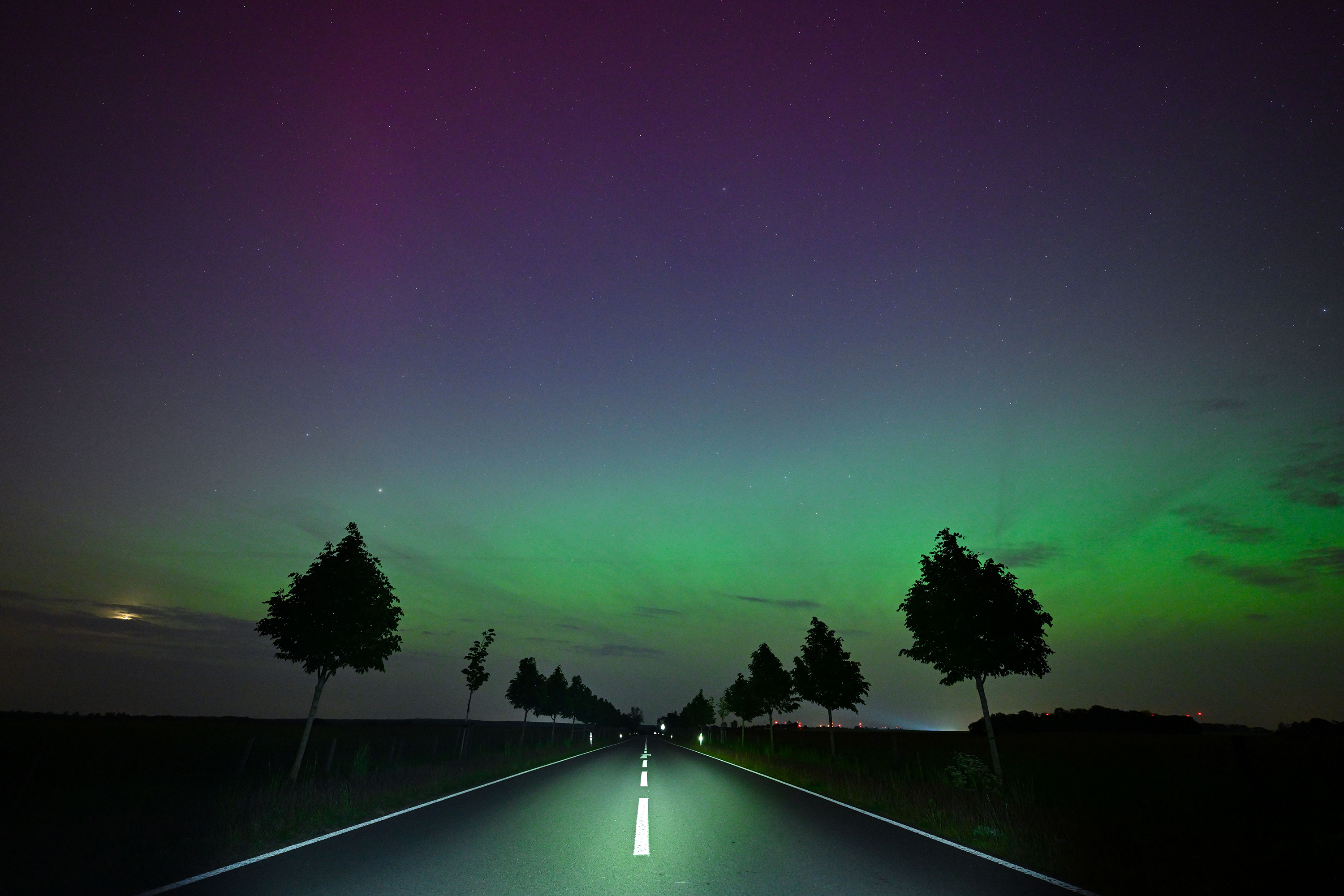
A series of solar flares and coronal mass ejections from the sun are creating dazzling auroras across the globe .
The rare solar storm may also disrupt communications. The last time a solar storm of this magnitude reached Earth was in October 2003, according to the National Oceanic and Atmospheric Administration's Space Weather Prediction Center.
See more photos of the aurora from tonight.
Behind dazzling aurora could lie “real danger,” Bill Nye the Science Guy says
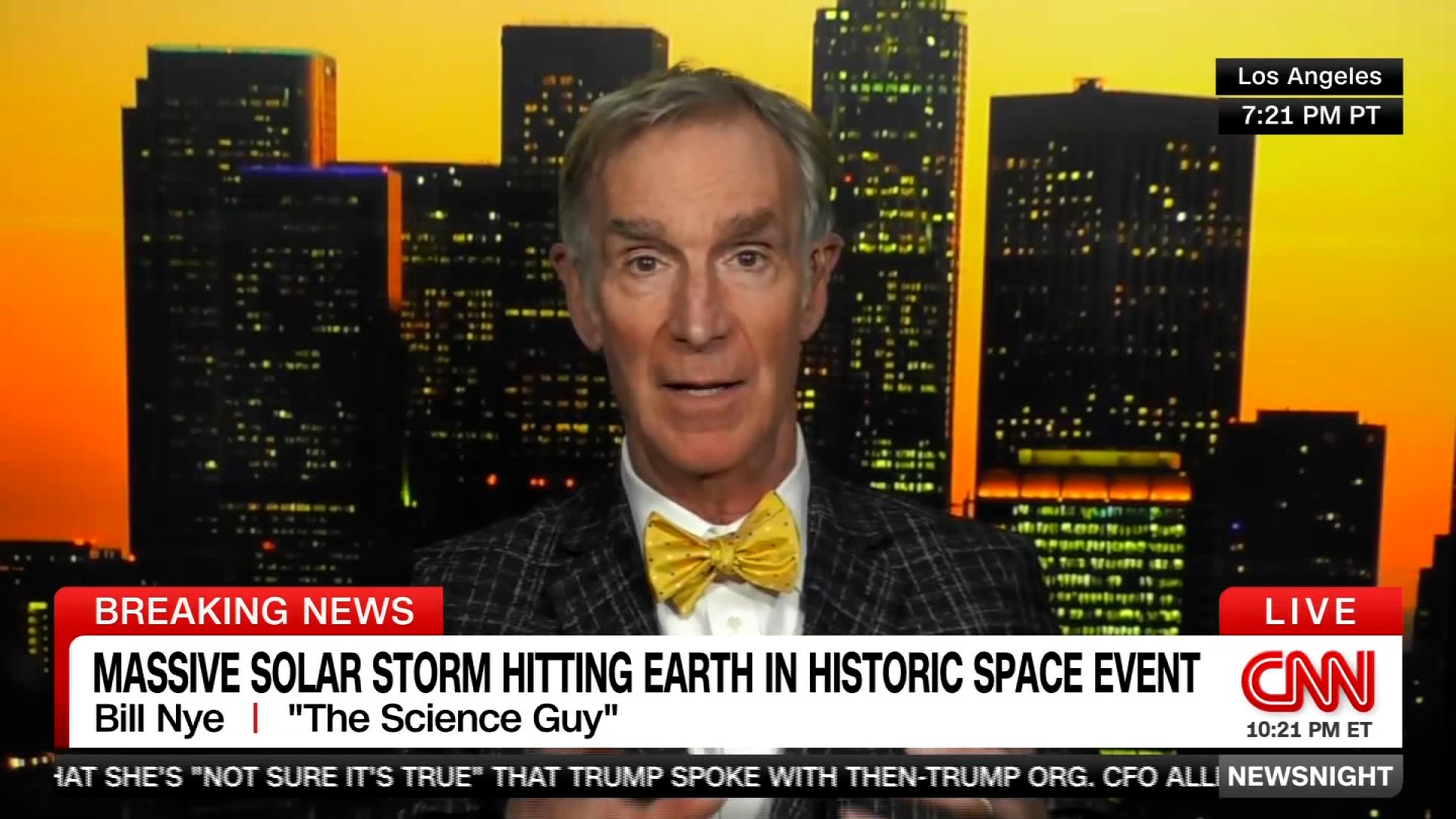
The massive solar storm could present “a real danger,” especially with the modern world relying so much on electricity, according to Bill Nye the Science Guy , a science educator and engineer.
Scientists are warning an increase in solar flares and coronal mass ejections from the sun have the potential to disrupt communication on Earth into the weekend. Solar flares can affect communications and GPS almost immediately because they disrupt Earth’s ionosphere, or part of the upper atmosphere. Energetic particles released by the sun can also disrupt electronics on spacecraft and affect astronauts without proper protection within 20 minutes to several hours.
In comparison to tonight's event, Nye drew comparisons with another incident in 1859, known as the Carrington Event, when telegraph communications were severely affected.
“The other thing, everybody, that is a real danger to our technological society, different from 1859, is how much we depend on electricity and our electronics and so on,” Nye said. "None of us really in the developed world could go very long without electricity."
He noted that there are systems in place to minimize the impact, but “stuff might go wrong,” stressing that not all transformers are equipped to withstand such a solar event.
“It depends on the strength of the event and it depends on how much of our infrastructures are prepared for this the sort of thing,” he said.
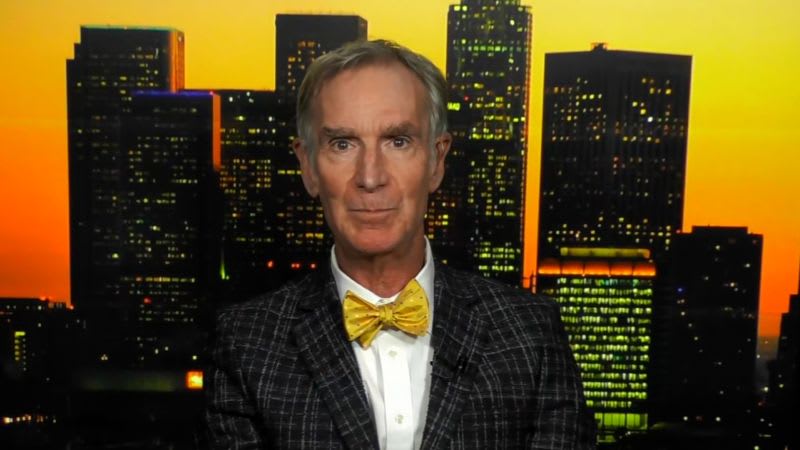
Bill Nye breaks down significance of the solar storm | CNN
This post has been updated with more details on solar flares' impact on electronics.
Here's where clouds will block the view of the northern lights in the US
From CNN's Angela Fritz
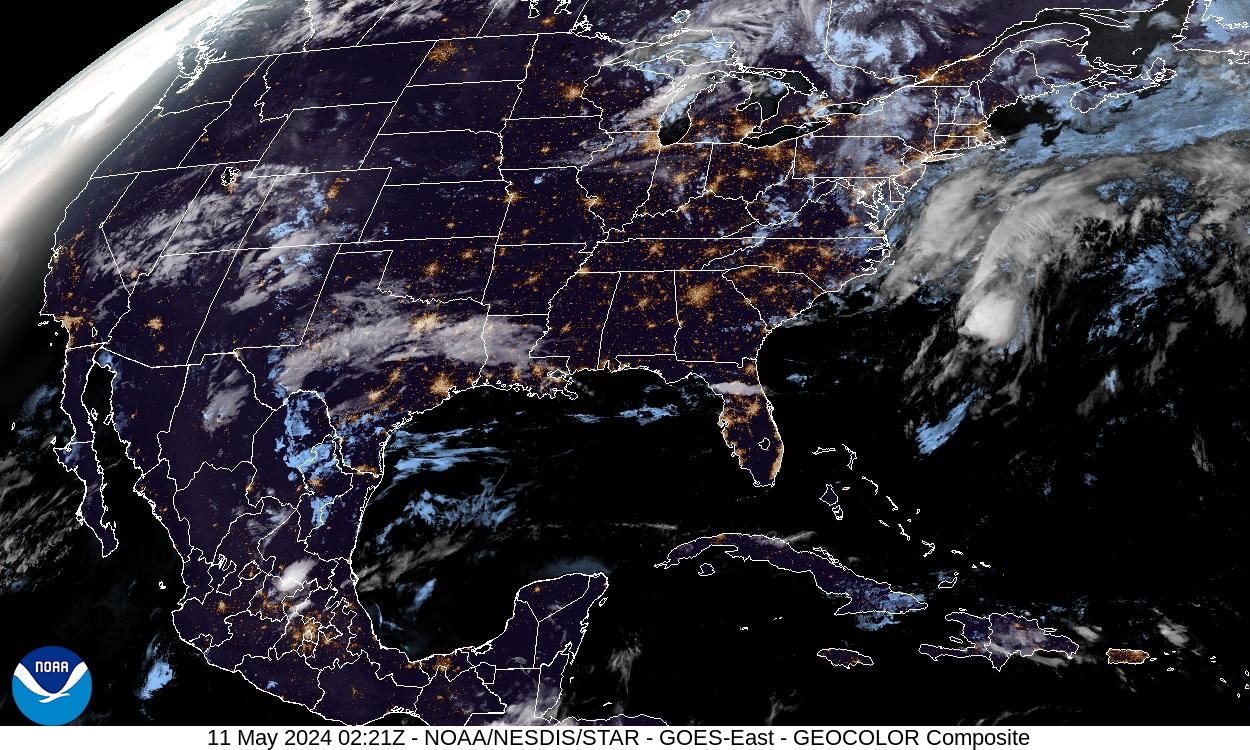
After an incredibly stormy week, most of the Lower 48 has clear skies to see the northern lights. But there are some areas where clouds and rainy weather are spoiling the view.
A deck of clouds is blocking the sky in the Northeast, from parts of Virginia into Maine, as an area of low pressure spins off the East Coast.
In the Midwest, the aurora will be hard to see through thick clouds in parts of Wisconsin, Michigan — including the Upper Peninsula — and Illinois.
A stripe of clouds is tracking across Texas, including Dallas-Forth Worth, and into Louisiana.
And in the Southwest, patchy clouds across the the Four Corners region could make the northern lights difficult to spot.
Aurora seen at least as far south as Georgia
Barely visible to the naked eye, the aurora can be seen in Atlanta in the 10 p.m. ET hour.
It is easier to see through photographs using a long exposure. The photos below, taken by CNN's Eric Zerkel and Emily Smith, used 3- and 10-second exposures.
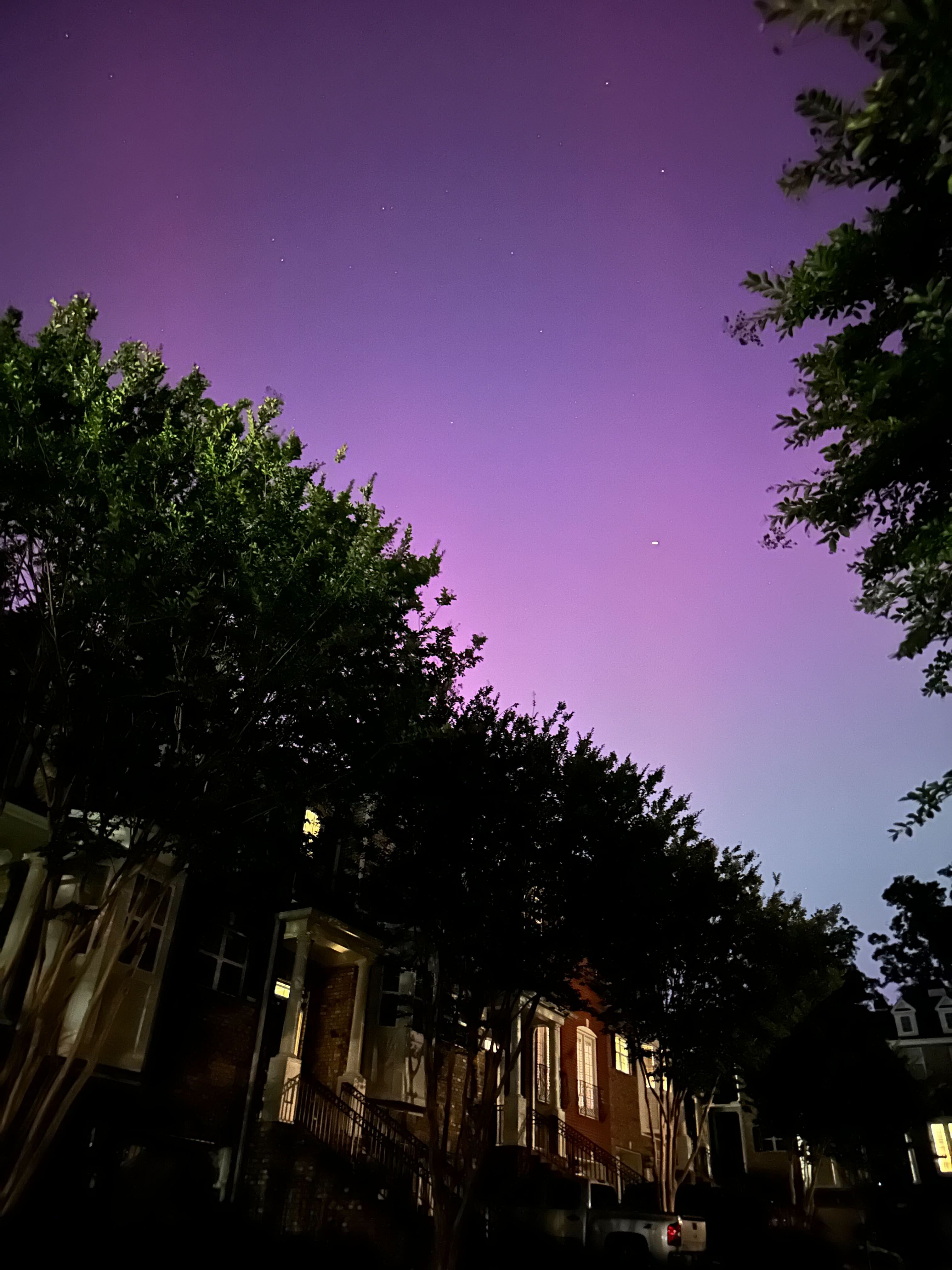
Please enable JavaScript for a better experience.

IMAGES
VIDEO
COMMENTS
Now consider the electric potential near a group of charges q 1, q 2, and q 3, as drawn in Figure 18.24. The electric potential is derived by considering the electric field. Electric fields follow the principle of superposition and can be simply added together, so the electric potential from different charges also add together.
Electric potential difference, also known as voltage, is the external work needed to bring a charge from one location to another location in an electric field. Electric potential difference is the change of potential energy experienced by a test charge that has a value of + 1. . .
electric potential, the amount of work needed to move a unit charge from a reference point to a specific point against an electric field.Typically, the reference point is Earth, although any point beyond the influence of the electric field charge can be used.. The diagram shows the forces acting on a positive charge q located between two plates, A and B, of an electric field E.
The electric potential, or voltage, is the difference in potential energy per unit charge between two locations in an electric field. When we talked about electric field, we chose a location and then asked what the electric force would do to an imaginary positively charged particle if we put one there. To find the electrical potential at a ...
Electric potential (also called the electric field potential, potential drop, the electrostatic potential) is defined as the amount of work energy needed per unit of electric charge to move the charge from a reference point to a specific point in an electric field. More precisely, the electric potential is the energy per unit charge for a test charge that is so small that the disturbance of ...
Electric Potential Difference. The electric potential difference between points A and B, VB − VA is defined to be the change in potential energy of a charge q moved from A to B, divided by the charge. Units of potential difference are joules per coulomb, given the name volt (V) after Alessandro Volta. 1V = 1J / C.
The Main Idea. Electric Potential Energy, like all forms of potential energy, is the potential for work to be done, in this case by the electric force.The Electric Potential (frequently referred to as voltage, from its SI unit, the Volt) is the Electric Potential Energy associated with the test charge (1 Coulomb), such that it depends only on the source, just as the electric field is related ...
V = kq r ⏟ point charge. where k is a constant equal to 9.0 × 109N ⋅ m2 / C2. The potential in Equation 7.4.1 at infinity is chosen to be zero. Thus, V for a point charge decreases with distance, whereas →E for a point charge decreases with distance squared: E = F qt = kq r2.
The electric potential due to a segment of charge dq at a distance x from P is k dq/x. The total electric potential is the algebraic sum of all the segments of the rod. Full size image. The electric potential dV at P due to this segment is given by: $$ dV=k { {dq}\over {x}}=k { {\lambda dx}\over {x}} $$.
The electric potential between the discharging electrode wire and the electrode plate is 10 kV. The diameters of the discharging electrode wires are 0.2 mm, 0.3 mm and 0.4 mm. As shown in this figure, the distributions of electric field have same trends for three diameters of the electrode wire. As the diameter of the wire decreases, there is a ...
The potential energy in Eq. 13.3 describes the potential energy of two charges, and therefore it is strictly dependent on which two charges we are considering. However, similarly to what we did in the previous chapter, when we defined the electric field created by a single source charge, it is convenient to also define a more general quantity to describe the electrostatic energy of a source ...
Figure 9.6.3: A typical electron gun accelerates electrons using a potential difference between two metal plates. The energy of the electron in electron volts is numerically the same as the voltage between the plates. For example, a 5000 V potential difference produces 5000 eV electrons. Definition: ELECTRON VOLT.
The passage of the charge Q causes the potential difference between the two plates to become equal to the potential difference of the battery, one plate thus becoming positively charged and the ...
[email protected]. [email protected]. PHY204 Lecture 9. [rln9] Electric Potential and Potential Energy: Application (1) Consider a point chargeQ=2mC xed at positionx =0. A particle with massm=2gand chargeq= 0.1mC is launched at positionx. 1. =10cm with velocity v.
Potential difference is the difference in electric potential of two points in the region of electric field. Solutions by analysis and computer calculation are presented. Discover the world's research
Nature's Electric Potential: A Systematic Review of the Role of Bioelectricity in Wound Healing and Regenerative Processes in Animals, Humans, and Plants ... it is concluded that the mapping of voltage patterns and the processes generating them is a promising future research focus, to probe three aspects: the role of wound/regeneration currents ...
1 Introduction. Variations in soil surface electric potential (EP), referred to as self-potential (SP) anomalies, are found in nature and are proposed to be associated with electrokinetic, electrochemical, thermoelectric, redox, and piezoelectric effects [Jouniaux et al., 2009].For instance, Sato and Mooney [] proposed that observed SP anomalies over ore bodies could be explained as spatially ...
Applying an external potential difference between two electrodes leads to a voltage drop in an ion conducting electrolyte. This drop is particularly large in poorly conducting electrolytes and for high currents. Measuring the electrolyte potential is relevant in electrochemistry, e.g., bipolar electrochemistry, ohmic microscopy, or contact glow discharge electrolysis. Here, we study the course ...
The Altmetric Attention Score is a quantitative measure of the attention that a research article has received online. Clicking on the donut icon will load a page at altmetric.com with additional details about the score and the social media presence for the given article. ... The electric potential-driven proton transfer/transport kinetic model ...
where m, v, q and x are particle mass, velocity, charge and position respectively; q = e for protons, q = −e for electrons; V is the electric potential at x; the subscript 0 denotes the initial state.Usually, the thermal velocity of a proton is much lower than its bulk velocity, so v in Equation is approximately equal to the proton bulk velocity; on the contrary, the electron thermal ...
The surfaces can be either conductors or insulators, and they must be capable of reaching a high enough voltage to generate a sufficient electric charge without being damaged. Triboelectric devices are still relatively in their infancy, with a number of researchers looking to further explore their potential.
Recent development and research on green energy sources can assure sustainable power supply for the islands. But unpredictable nature and high dependency on weather conditions are the main limitations of renewable energy sources. ... The potential that anneals the electric field at the surface of the probe (to simulate its principle of ...
Monitoring the electric potential (EP) response induced by coal deformation and rupture is essential to the safety of underground engineering under extreme load and stress wave disturbance conditions. In this study, the split Hopkinson pressure bar and EP acquisition systems were utilized for EP testing of coal under stress waves. The EP response under stress waves was investigated, the ...
The electric potential gradually decreases from the needle to the receiving plate, so the distribution of the electric potential field in the model is consistent with the actual situation and has certain rules. ... In this research, we propose a multi-needle electrospinning technique based on cross-wind field assistance system, and investigate ...
Transverse-electric (TE) surface plasmon polaritons are unique eigenmodes of a homogeneous graphene layer that are tunable with the chemical potential and temperature. However, as their dispersion curve spectrally lies below the light line, they cannot be resonantly excited by an externally incident wave. Here, we propose a way of exciting the TE modes and tuning their peaks in the ...
Aurora seen in Atlanta area around 10:30 p.m. ET. (Emily Smith/CNN) A stunning aurora, caused by a severe geomagnetic storm, is painting the sky shades of pink, purple and green as it spreads into ...
Integrating urban bus parking lots with photovoltaics is an important solution to promote carbon neutrality and net zero emissions in transportation systems. Except for the research on the integration of photovoltaics with car parking lots and bus roofs, not much attention has been paid to photovoltaic bus parking lots so far. We develop a new methodology for evaluating the potential of urban ...