Universality
When we started talking about critical opalescence, we spoke of water and carbon dioxide and the gas-liquid transition. The experiment described something different – two different liquids going through a “dissolved/not-dissolved” transition. But everything we understood about critical phenomena from the liquid-gas transition seemed to work in the experiment.
The connection is deeper than that. If you look at the way response functions like compressibility get big around the critical point (how fast as you bring the temperature close to the critical point), you find that you end up with the same numbers for lots of very diverse situations.
As scientists, we have to take one more important step: we have to be a little more concrete in the description of “sameness”. This requires a little math, but it’s totally worth the effort. So here it goes: things get big (diverge) around the critical temperature as
- As T gets close to T c , the function diverges or “blows up” (gets really big).
- There are three lines below corresponding to three different values of the critical exponent , x. Match the line to the critical exponent.
- The critical exponent controls how fast the function blows up. The bigger the exponent, the faster the blow up. A gas-liquid critical point, like that for water or carbon dioxide, is associated with a bunch of different critical exponents – one for isothermal compressibility, one for heat capacity, and so on. We just recycle the same function with a different x .
The remarkable thing about critical phenomena is that a bunch of different kinds of quantities – response functions like compressibility and others – follow this rule close to the critical temperature. There might be a different critical exponent for each different kind of quantity, but the same kind of expression represents the behavior of all of these quantities.
The even more remarkable thing is that the critical exponents – the x 's – for totally different systems are the same .

Ask yourself…
- What happens at the critical point that is always the same?
- What all of these situations have in common are fluctuations about an average state (temperature and pressure). Right around the critical point, these fluctuations become magnified, and control the way the system behaves. Because the fluctuations grow in the same way – whether they are fluctuations of average density or magnetization or any other order parameter – the same rate of growth appears for all of these systems….WOW!
….or something like that – it’s a little more complicated. It turns out that the critical behavior is not always the same, but it doesn’t depend so much on the identity of the substance at play – more on its dimensionality and number of components.
To gain further understanding, we need to open up this black box of fluctuations to figure out what is happening around the critical point that makes them grow so large.
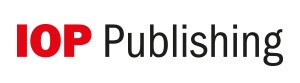
We apologize for the inconvenience...
To ensure we keep this website safe, please can you confirm you are a human by ticking the box below.
If you are unable to complete the above request please contact us using the below link, providing a screenshot of your experience.
https://ioppublishing.org/contacts/
Experimental Critical-Exponent Values for Fluids
- Published: 08 October 2009
- Volume 137 , pages 857–877, ( 2009 )
Cite this article
- Jan V. Sengers 1 &
- Joseph G. Shanks 2
847 Accesses
159 Citations
Explore all metrics
After a review of the history and an assessment of the current status of the subject, we present light-scattering data to determine the critical correlation-function exponents for an aqueous electrolyte solution yielding the experimental values γ =1.238±0.012, ν =0.629±0.003, η =0.032±0.013. We conclude the paper with some comments concerning the temperature dependence of non-asymptotic effective critical-exponent values of fluids.
This is a preview of subscription content, log in via an institution to check access.
Access this article
Subscribe and save.
- Get 10 units per month
- Download Article/Chapter or eBook
- 1 Unit = 1 Article or 1 Chapter
- Cancel anytime
Price includes VAT (Russian Federation)
Instant access to the full article PDF.
Rent this article via DeepDyve
Institutional subscriptions
Similar content being viewed by others
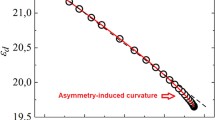
Liquid-liquid criticality in the dielectric constant and refractive index: A perspective
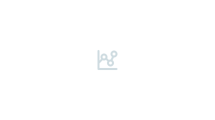
Critical Crossover Functions for Simple Fluids: Towards the Crossover Modelling Uniqueness
Fluctuation theory of critical phenomena in fluids.
Fairbank, W.M., Buckingham, M.J., Kellers, C.F.: In: Dillinger, J.R. (ed.) Proc. 5th International Conference on Low Temperature Physics, p. 50. University of Wisconsin Press, Madison (1957)
Google Scholar
Buckingham, M.J., Fairbank, W.M.: In: Gorter, C.J. (ed.) Progress in Low Temperature Physics, vol. III, p. 86. North Holland, Amsterdam (1961)
Onsager, L.: Phys. Rev. 65 , 117–149 (1944)
MATH ADS MathSciNet Google Scholar
Bagataskii, M.I., Voronel, A.V., Gusak, V.G.: Z. Eksp. Teor. Fiz. 43 , 728–729 (1962) [English translation: Sov. Phys. JETP 16 , 517–518 (1963)]
Voronel, A.V., Chaskin, Yu., Popov, V.A., Simkon, V.G.: Z. Eksp. Teor. Fiz. 45 , 828–830 (1963) [English translation: Sov. Phys. JETP 18 , 568–569 (1964)]
Sengers, J.V., Michels, A.: In: Masi, J.F., Tsai, D.H. (eds.) Proc. 2nd Symposium on Thermophysical Properties, pp. 434–440. American Society of Mechanical Engineers, New York (1962)
Moldover, M.R., Little, W.A.: Phys. Rev. Lett. 15 , 54–56 (1965)
ADS Google Scholar
Green, M.S., Sengers, J.V. (eds.): Proceedings of a Conference Held in Washington, D.C., April 1965. National Bureau of Standards Miscellaneous Publ., vol. 273. U.S. Government Printing Office, Washington (1966)
Fisher, M.E.: In: Green, M.S., Sengers, J.V. (eds.) Proceedings of a Conference Held in Washington, D.C., April 1965. National Bureau of Standards Miscellaneous Publ., vol. 273, pp. 21–26. U.S. Government Printing Office, Washington (1966)
Kadanoff, L.P.: In: Green, M.S. (ed.) Critical Phenomena. Varenna Lectures, Course LI, pp. 100–117. Academic Press, New York (1971)
Fisher, M.E.: Rev. Mod. Phys. 46 , 597–616 (1974)
Kadanoff, L.P.: In: Domb, C., Green, M.S. (eds.) Phase Transitions and Critical Phenomena, vol. 5a, pp. 1–34. Academic Press, New York (1976)
Wilson, K.G.: Sci. Am. 241 , 158–179 (1979)
Article Google Scholar
Wilson, K.G.: Rev. Mod. Phys. 55 , 583–600 (1983)
Fisher, M.E.: Rev. Mod. Phys. 70 , 653–681 (1998)
Heller, P.: Rep. Prog. Phys. XXX (II), 731–826 (1967)
Kadanoff, L.P., Götze, W., Hamblen, D., Hecht, R., Lewis, E.A., Palciauskas, V.V., Rayl, M., Swift, J.: Rev. Mod. Phys. 39 , 395–431 (1967)
Egelstaff, P.A., Ring, J.W.: In: Temperley, H.N.V., Rowlinson, J.S., Rushbrooke, G.S. (eds.) Physics of Simple Liquids, pp. 253–297. North-Holland, Amsterdam (1968)
Chu, B.: Ber. Bunsenges. Phys. Chem. 76 , 202–215 (1972)
Anisimov, M.A.: Usp. Fiz. Nauk 114 , 249–294 (1974) [English translation: Sov. Phys. Usp. 17 , 722–744 (1975)]
Wallace, B., Meyer, H.: Phys. Rev. A 2 , 1563–1575 (1970)
Weber, L.: Phys. Rev. A 2 , 2379–2388 (1970)
Levelt Sengers, J.M.H., Sengers, J.V.: Phys. Rev. A 12 , 2622–2627 (1975)
Balzarini, D., Ohrn, K.: Phys. Rev. Lett. 29 , 840–842 (1972)
Schmidt, E.H.W.: In: Green, M.S., Sengers, J.V. (eds.) Proceedings of a Conference Held in Washington, D.C., April 1965. National Bureau of Standards Miscellaneous Publ., vol. 273, pp. 13–20. U.S. Government Printing Office, Washington (1966)
Moldover, M.R., Sengers, J.V., Gammon, R.W., Hocken, R.J.: Rev. Mod. Phys. 51 , 79–99 (1979)
Green, M.S., Vicentini-Missoni, M., Levelt Sengers, J.M.H.: Phys. Rev. Lett. 18 , 1113–1117 (1967)
Widom, B.: J. Chem. Phys. 43 , 3898–3905 (1965)
Wilcox, L.R., Balzarini, D.: J. Chem. Phys. 48 , 753–763 (1968)
Hocken, R., Moldover, M.R.: Phys. Rev. Lett. 37 , 29–32 (1976)
Levelt Sengers, A., Hocken, R., Sengers, J.V.: Phys. Today 30 (12), 42–51 (1977)
Wegner, F.J.: Phys. Rev. B 5 , 4529–4536 (1972)
Greer, S.C.: Phys. Rev. A 14 , 1770–1780 (1976)
Wims, A.M., McIntyre, D., Hynne, F.: J. Chem. Phys. 50 , 616–620 (1969)
Camp, W.J., Saul, D.M., Van Dyke, J.P., Wortis, M.: Phys. Rev. B 14 , 3990–4001 (1976)
Chang, R.F., Burstyn, H., Sengers, J.V., Bray, A.J.: Phys. Rev. Lett. 37 , 1481–1484 (1976)
Chang, R.F., Burstyn, H., Sengers, J.V.: Phys. Rev. A 19 , 866–882 (1979)
Baker, G.A. Jr., Nickel, B.G., Green, M.S., Meiron, D.I.: Phys. Rev. Lett. 36 , 1351–1354 (1976)
Levy, M., Le Guillou, J.-C., Zinn-Justin, J. (eds.): Phase Transitions Cargèse 1980. Plenum, New York (1982)
Nickel, B.G.: In: Levy, M., Le Guillou, J.-C., Zinn-Justin, J. (eds.) Phase Transitions Cargèse 1980, pp. 291–324. Plenum, New York (1982)
Moldover, M.R.: Phys. Rev. 182 , 342–352 (1969)
Lipa, J.A., Edwards, C., Buckingham, M.J.: Phys. Rev. Lett. 25 , 1086–1090 (1970)
Lai, C.C., Chen, S.H.: Phys. Lett. A 41 , 259–260 (1972)
Thiel, D., Chu, B., Stein, A., Allen, G.: J. Chem. Phys. 62 , 3689–3711 (1975)
Cannell, D.S.: Phys. Rev. A 12 , 225–231 (1975)
Lipa, J.A., Edwards, C., Buckingham, M.J.: Phys. Rev. A 15 , 778–789 (1977)
Jacobs, D.T., Anthony, D.J., Mockler, R.C., O’Sullivan, W.J.: Chem. Phys. 20 , 219–226 (1977)
Nakata, M., Dobashi, T., Kuwara, N., Kaneko, M., Chu, B.: Phys. Rev. A 18 , 2683–2688 (1978)
Beysens, D.: J. Chem. Phys. 71 , 2557–2565 (1979)
Beysens, D., Bourgou, A.: Phys. Rev. A 19 , 2407–2420 (1979)
Balzarini, D., Burton, M.: Can. J. Phys. 57 , 1516–1517 (1979)
Pittman, C., Doiron, T., Meyer, H.: Phys. Rev. B 20 , 3678–3689 (1979)
Nagarajan, N., Kumar, A., Gopal, E.S.R., Greer, S.C.: J. Phys. Chem. 84 , 2883–2887 (1980)
Bloemen, E., Thoen, J., Van Dael, W.: J. Chem. Phys. 73 , 4628–4635 (1980)
Thijsse, B.J.: J. Chem. Phys. 74 , 4678–4692 (1981)
Güttinger, H., Cannell, D.S.: Phys. Rev. A 24 , 3188–3201 (1981)
Beysens, D., Bourgou, A., Calmettes, P.: Phys. Rev. A 26 , 3589–3609 (1982)
Beysens, D.: In: Levy, M., Le Guillou, J.-C., Zinn-Justin, J. (eds.) Phase Transitions Cargèse 1980, pp. 25–62. Plenum, New York (1982)
Moldover, M.R.: In: Levy, M., Le Guillou, J.-C., Zinn-Justin, J. (eds.) Phase Transitions Cargèse 1980, pp. 63–94. Plenum, New York (1982)
Sengers, J.V.: In: Levy, M., Le Guillou, J.-C., Zinn-Justin, J. (eds.) Phase Transitions Cargèse 1980, pp. 95–135. Plenum, New York (1982)
Ahlers, G.: In: Levy, M., Le Guillou, J.-C., Zinn-Justin, J. (eds.) Phase Transitions Cargèse 1980, pp. 1–23. Plenum, New York (1982)
Anisimov, M.A., Voronel, A.V., Ovodova, T.M.: Z. Eksp. Teor. Fiz. 61 , 1092–1100 (1971) [English translation: Sov. Phys. JETP 34 , 583–587 (1972)]
Anisimov, M.A., Gorodetskii, E.E., Shmakov, N.G.: Z. Eksp. Teor. Fiz. 63 , 2165–2179 (1972) [English translation: Sov. Phys. JETP 36 , 1143–1150 (1973)]
Anisimov, M.A., Berestov, A.T., Voronov, V.P., Kiachenko, Yu.F., Koval’chuk, B.A., Malyshev, V.M., Smirnov, V.A.: Z. Eksp. Teor. Fiz. 76 , 1661–1669 (1979) [English translation: Sov. Phys. JETP 49 , 844–848 (1979)]
Anisimov, M.A.: Critical Phenomena in Liquids and Liquid Crystals. Gordon and Breach, Philadelphia (1991)
Greer, S.C., Moldover, M.R.: Ann. Rev. Phys. Chem. 32 , 233–265 (1981)
Corti, M., Degiorgio, V.: Phys. Rev. Lett. 45 , 1045–1048 (1980)
Corti, M., Degiorgio, V., Zulauf, M.: Phys. Rev. Lett. 48 , 1617–1620 (1982)
Corti, M., Minero, C., Degiorgio, V.: J. Phys. Chem. 88 , 309–317 (1984)
Degiorgio, V., Piazza, R., Corti, M., Minero, C.: J. Chem. Phys. 82 , 1025–1031 (1985)
Corti, M., Degiorgio, V.: Phys. Rev. Lett. 55 , 2005–2008 (1985)
Fisher, M.E.: Phys. Rev. Lett. 57 , 1911–1914 (1986)
Dietler, G., Cannell, D.S.: Phys. Rev. Lett. 60 , 1852–1855 (1988)
Hamano, K., Kaneko, T., Fukuhara, K., Kuwahara, N.: Int. J. Thermophys. 10 , 389–396 (1989)
Hamano, K., Kuwahara, N., Mitsushima, I., Kubota, K., Kamura, T.: J. Chem. Phys. 94 , 2172–2175 (1991)
Rouch, J., Tartaglia, P., Safouane, A., Chen, S.H.: Phys. Rev. A 40 , 2013–2021 (1989)
Singh, R.R., Pitzer, K.S.: J. Am. Chem. Soc. 110 , 8723–8724 (1988)
Singh, R.R., Pitzer, K.S.: J. Chem. Phys. 92 , 6775–6778 (1990)
Zhang, K.C., Briggs, M.E., Gammon, R.W., Levelt Sengers, J.M.H.: J. Chem. Phys. 97 , 8692–8697 (1992)
Zhang, K.C., Briggs, M.E., Gammon, R.W., Levelt Sengers, J.M.H.: J. Chem. Phys. 105 , 4397 (1996)
Pitzer, K.S.: Acc. Chem. Res. 23 , 333–338 (1990)
Fisher, M.E.: J. Stat. Phys. 75 , 1–36 (1994)
Wiegand, S., Levelt Sengers, J.M.H., Zhang, K.J., Briggs, M.E., Gammon, R.W.: J. Chem. Phys. 106 , 2777–2781 (1997)
Wiegand, S., Briggs, M.E., Levelt Sengers, J.M.H., Kleemeier, M., Schröer, W.: J. Chem. Phys. 109 , 9038–9051 (1998)
Kostko, A.F., Anisimov, M.A., Sengers, J.V.: Phys. Rev. E 70 , 026118 (2004)
Anisimov, M.A., Jacob, J., Kumar, A., Agayan, V.A., Sengers, J.V.: Phys. Rev. Lett. 85 , 2336–2339 (2000)
Schröer, W., Wiegand, S., Weingärtner, H.: Ber. Bunsenges. Phys. Chem. 97 , 975–982 (1993)
Wiegand, S., Kleemeier, M., Schröder, J.M., Schröer, W., Weingärtner, H.: Int. J. Thermophys. 15 , 1045–1056 (1994)
Kleemeier, M., Schröer, W., Weingärtner, H.: J. Mol. Liq. 73–74 , 501–511 (1997)
Kleemeier, M., Wiegand, S., Schröer, W.: J. Chem. Phys. 110 , 3085–3099 (1999)
Wagner, M., Stanga, O., Schröer, W.: Phys. Chem. Chem. Phys. 5 , 1225–1234 (2003)
Wagner, M., Stanga, O., Schröer, W.: Phys. Chem. Chem. Phys. 6 , 580–589 (2004)
Schröer, W., Wagner, M., Stanga, O.: J. Mol. Liq. 127 , 2–9 (2006)
Japas, M.L., Levelt Sengers, J.M.H.: J. Phys. Chem. 94 , 5361–5368 (1990)
Bonetti, M., Bagnuls, C., Bervillier, C.: J. Chem. Phys. 107 , 550–561 (1997)
Gutkowski, K.I., Bianchi, H.L., Japas, M.L.: J. Chem. Phys. 118 , 2808–2814 (2003)
Van Roie, B., Pitsi, G., Thoen, J.: J. Chem. Phys. 119 , 8047–8051 (2003)
Madhavan Unni, P.K.: J. Chem. Phys. 124 , 054505 (2006)
Martín, A., López, I., Monroy, F., Casielles, A.G., Ortega, F., Rubio, R.G.: J. Chem. Phys. 101 , 6874–6879 (1994)
Levelt Sengers, J.M.H., Harvey, A.H., Wiegand, S.: In: Sengers, J.V., Kayser, R.F., Peters, C.J., White, H.J. Jr. (eds.) Equations of State of Fluids and Fluid Mixtures, pp. 805–847. Elsevier, Amsterdam (2000)
Fisher, M.E.: In: Hahne, F.J.W. (ed.) Critical Phenomena. Lecture Notes in Physics, vol. 186, pp. 1–139. Springer, Berlin (1983)
Fisher, M.E.: J. Math. Phys. 5 , 944–962 (1964)
Fisher, M.E., Burford, R.J.: Phys. Rev. 156 , 583–622 (1967)
Pelissetto, A., Vicari, E.: Phys. Rep. 368 , 549–727 (2002)
Lee, T.D., Yang, C.N.: Phys. Rev. 87 , 410–419 (1952)
Fisher, M.E.: Rep. Prog. Phys. 30 (II), 615–730 (1967)
Fisher, M.E., Orkoulas, G.: Phys. Rev. Lett. 85 , 696–699 (2000)
Orkoulas, G., Fisher, M.E., Üstün, C.: J. Chem. Phys. 113 , 7530–7545 (2000)
Orkoulas, G., Fisher, M.E., Panagiotopoulos, A.Z.: Phys. Rev. E 63 , 051507 (2001)
Kim, Y.C., Fisher, M.E., Orkoulas, G.: Phys. Rev. E 67 , 061506 (2003)
Kim, Y.C., Fisher, M.E.: Chem. Phys. Lett. 414 , 185–192 (2005)
Anisimov, M.A., Wang, J.T.: Phys. Rev. Lett. 13 , 025703 (2006)
Wang, J.T., Anisimov, M.A.: Phys. Rev. E 75 , 051107 (2007)
Cerdeiriña, C.A., Anisimov, M.A., Sengers, J.V.: Chem. Phys. Lett. 424 , 414–419 (2006)
Wang, J.T., Cerdeiriña, C.A., Anisimov, M.A., Sengers, J.V.: Phys. Rev. E 77 , 031127 (2008)
Behnejad, H., Sengers, J.V., Anisimov, M.A., In: Goodwin, A.H., Sengers, J.V., Peters, C.J. (eds.) Applied Thermodynamics. Royal Society of Chemistry, Cambridge (in press)
Mermin, N.D.: Phys. Rev. Lett. 26 , 957–959 (1971)
Rehr, J.J., Mermin, N.D.: Phys. Rev. A 8 , 472–480 (1973)
Wheeler, J.C.: Ann. Rev. Phys. Chem. 28 , 411–443 (1977)
Kumar, A., Krishnamurthy, H.R., Gopal, E.S.R.: Phys. Rep. 98 , 57–143 (1983)
Sanchez, G., Meichle, M., Garland, C.W.: Phys. Rev. A 28 , 1647–1653 (1983)
Thoen, J., Hamelin, J., Bose, T.K.: Phys. Rev. E 53 , 6264–6270 (1996)
Fleweling, A.C., DeFonseka, R.J., Khaleeli, N., Partee, J., Jacobs, D.T.: J. Chem. Phys. 104 , 8048–8057 (1996)
Rebillot, P.F., Jacobs, D.T.: J. Chem. Phys. 109 , 4009–4014 (1998)
Haupt, A., Straub, J.: Phys. Rev. E 59 , 1795–1802 (1999)
Nowicki, A.W., Ghosh, M., McClellan, S.M., Jacobs, D.T.: J. Chem. Phys. 114 , 4625–4633 (2001)
Oby, E.R., Jacobs, D.T.: J. Chem. Phys. 114 , 4918–4921 (2001)
Utt, N., Lehman, S.Y., Jacobs, D.T.: J. Chem. Phys. 127 , 104505 (2007)
Cerdeiriña, C.A., Troncoso, J., Carballo, E., Romaní, L.: Phys. Rev. E 66 , 031507 (2002)
Troncoso, J., González-Salgado, D., Cerdeiriña, C.A., Carballo, E., Romaní, L.: Phys. Rev. E 71 , 021503 (2005)
Handschy, M.A., Mockler, R.C., O’Sullivan, W.J.: Chem. Phys. Lett. 76 , 172–174 (1980)
Shelton, J., Balzarini, D.A.: Can. J. Phys. 59 , 934–935 (1981)
de Bruyn, J.R., Balzarini, D.A.: Phys. Rev. B 39 , 9243–9251 (1989)
Närger, U., Balzarini, D.A.: Phys. Rev. B 39 , 9330–9335 (1989)
Pestak, M.W., Chan, M.H.W.: Phys. Rev. B 30 , 274–288 (1984)
Houessou, C., Guenoun, P., Gastaud, R., Perrot, F., Beysens, D.: Phys. Rev. A 32 , 1818–1833 (1985)
Vani, V., Guha, S., Gopal, E.S.R.: J. Chem. Phys. 84 , 3999–4007 (1986)
Biswas, S.N., ten Seldam, C.A.: Fluid Phase Equilib. 47 , 67–75 (1989)
Biswas, S.N., ten Seldam, C.A., Bominaar, S.A.R.C., Trappeniers, N.J.: Fluid Phase Equilib. 49 , 1–7 (1989)
Jacobs, D.T.: J. Phys. Chem. 86 , 1895–1898 (1982)
Ploplis, A.C., Wardwell, P.S., Jacobs, D.T.: J. Phys. Chem. 90 , 4676–4678 (1986)
Andrew, W.V., Khoo, T.B.K., Jacobs, D.T.: J. Chem. Phys. 85 , 3985–3991 (1986)
Jacobs, D.T., Kuhl, D.E., Selby, C.E.: J. Chem. Phys. 105 , 586–597 (1996)
Bouanz, M., Beysens, D.: Chem. Phys. Lett. 231 , 105–110 (1994)
Bonetti, M., Oleinikova, A., Bervillier, C.: J. Phys. Chem. B 101 , 2164–2173 (1997)
Oleinikova, A., Bonetti, M.: Chem. Phys. Lett. 299 , 417–422 (1999)
An, X., Zhao, H., Jiang, F., Mao, C., Shen, W.: J. Chem. Thermodyn. 29 , 1047–1054 (1997)
An, X., Zhao, H., Jiang, F., Shen, W.: J. Chem. Thermodyn. 30 , 21–26 (1998)
An, X., Jiang, F., Zhao, H., Chen, C., Shen, W.: J. Chem. Thermodyn. 30 , 751–760 (1998)
An, X., Li, P., Zhao, H., Shen, W.: J. Chem. Thermodyn. 30 , 1049–1059 (1998)
An, X., Jiang, F., Zhao, H., Shen, W.: J. Chem. Thermodyn. 30 , 1181–1190 (1998)
Zhou, C., An, X., Jiang, F., Zhao, H., Shen, W.: J. Chem. Thermodyn. 31 , 615–626 (1999)
Tang, K., Zhou, C., An, X., Shen, W.: J. Chem. Thermodyn. 31 , 943–954 (1999)
Oleinikova, A., Weingärtner, H.: Chem. Phys. Lett. 319 , 119–124 (2000)
Wang, N., Mao, C., Lu, R., Peng, X., An, X., Shen, W.: J. Chem. Thermodyn. 38 , 264–271 (2006)
Khairulin, R.A., Stankus, S.V., Gruzdev, V.A.: Int. J. Thermophys. 28 , 1245–1254 (2007)
Chen, S.-H., Lai, C.-C., Rouch, J., Tartaglia, P.: Phys. Rev. A 27 , 1086–1095 (1983)
Ostwald, U., Belkoura, L., Jungk, M., Woermann, D.: Ber. Bunsenges. Phys. Chem. 88 , 635–642 (1984)
Jacobs, D.T.: Phys. Rev. A 33 , 2605–2611 (1986)
ADS MathSciNet Google Scholar
DaMore, L.W., Jacobs, D.T.: J. Chem. Phys. 97 , 464–469 (1992)
Lytle, A., Jacobs, D.T.: J. Chem. Phys. 120 , 5709–5716 (2004)
Anisimov, M., Thoen, J.: In: Wilhelm, E., Letcher, T.M. (eds.) Heat Capacities: Liquids and Vapours. Royal Society of Chemistry, Cambridge (in press)
Barmatz, M., Hahn, I., Lipa, J.A., Duncan, R.V.: Rev. Mod. Phys. 79 , 1–52 (2007)
Dobashi, T., Nakata, M., Kaneko, M.: J. Chem. Phys. 72 , 6685–6691 (1980)
Stafford, S.G., Ploplis, A.C., Jacobs, D.T.: Macromolecules 23 , 470–475 (1990)
Shen, W., Smith, G.R., Knobler, C.M., Scott, R.L.: J. Phys. Chem. 95 , 3376–3379 (1991)
Kuwahara, N., Sato, H., Kubota, K.: Phys. Rev. E 48 , 3176–3179 (1993)
An, X.-Q., Shen, W.-G., Xia, K.-Q.: J. Chem. Phys. 107 , 2060–2065 (1997)
An, X., Jiang, F., Chen, C., Shen, W.: Chem. Phys. Lett. 282 , 403–408 (1998)
An, X., Xia, K.-Q., Shen, W., Qiu, X.-L.: J. Chem. Phys. 111 , 8289–8301 (1999)
Zhou, C.-S., An, X.-Q., Xia, K.-Q., Yin, X.-L., Shen, W.-G.: J. Chem. Phys. 117 , 4557–4563 (2002)
Jacobs, D.T., Braganza, C.I., Brinck, A.P., Cohen, A.B., Lightfoot, M.A., Locke, C.J., Suddendorf, S.J., Timmers, H.R., Triplett, A.L., Venkataraman, N.L., Wellons, M.T.: J. Chem. Phys. 127 , 124905 (2007)
Anisimov, M.A., Kostko, A.F., Sengers, J.V.: Phys. Rev. E 65 , 051805 (2002)
Anisimov, M.A., Sengers, J.V.: Mol. Phys. 103 , 3061–3070 (2005)
Anisimov, M.A., Kostko, A.F., Sengers, J.V., Yudin, I.K.: J. Chem. Phys. 123 , 164901 (2005)
Shimanskaya, E.T., Shimansky, Yu.I., Oleinikova, A.V.: Int. J. Thermophys. 17 , 641–649 (1996)
Shimansky, Yu.I., Shimanskaya, E.T.: Int. J. Thermophys. 17 , 651–662 (1996)
Shimanskaya, E.T., Shimansky, Yu.I.: High Temp.-High Press. 29 , 509–518 (1997)
Shimansky, Yu.I., Shimanskaya, E.T.: High Temp.-High Press. 30 , 635–643 (1998)
Fameli, N., Balzarini, D.A.: Phys. Rev. E 75 , 064203 (2007)
Wagner, W., Kurzeja, N., Pieperbeck, B.: Fluid Phase Equilib. 79 , 151–174 (1992)
Kurzeja, N., Tielkes, Th., Wagner, W.: Int. J. Thermophys. 20 , 531–561 (1999)
Guder, C., Wagner, W.: J. Phys. Chem. Ref. Data 38 , 33–94 (2009)
Ivanov, D.Yu., Makarevich, L.A., Sokolova, O.N.: ZhETF Pis. Red. 20 , 272–276 (1974) [English translation: JETP Lett. 20 , 121–122 (1974)]
Makarevich, L.A., Sokolova, O.N., Rozen, A.M.: Z. Eksp. Teor. Fiz. 67 , 615–620 (1974) [English translation: Sov. Phys.-JETP 40 , 305–307 (1975)]
Ivanov, D.Yu.: Dokl. Akad. Nauk 383 , 478–481 (2002)
Ivanov, D.Yu.: Critical Behavior of Non-Ideal Systems. Wiley-VCH, Weinheim (2008)
MATH Google Scholar
Lecoutre, C., Garrabos, Y., Georgin, E., Palencia, E., Beysens, D.: Int. J. Thermophys. 30 , 810–832 (2009)
Wagner, W.: In: Proc. 15th International Conference on the Properties of Water and Steam. VDI-Gesellschaft (2008), 11 pp. ISBN 978-3-931384-64-7
Hohenberg, P.C., Barmatz, M.: Phys. Rev. A 6 , 289–313 (1972)
Onuki, A., Kawasaki, K.: Ann. Phys. 121 , 456–527 (1979)
MATH ADS Google Scholar
Onuki, A., Yamazaki, K., Kawasaki, K.: Ann. Phys. 131 , 217–242 (1981)
Onuki, A.: Phase Transition Dynamics. Cambridge Univ. Press, Cambridge (2002), Chap. 11
Beysens, D., Gbadamassi, M.: Phys. Rev. A 22 , 2250–2261 (1980)
Beysens, D., Gbadamassi, M., Moncef-Bouanz, B.: Phys. Rev. A 28 , 2491–2509 (1983)
Sengers, J.V., van Leeuwen, J.M.J.: Physica A 116 , 345–367 (1982)
van Leeuwen, J.M.J., Sengers, J.V.: Physica A 128 , 99–131 (1984)
van Leeuwen, J.M.J., Sengers, J.V.: Physica A 132 , 207–232 (1985)
Sengers, J.V., van Leeuwen, J.M.J.: Int. J. Thermophys. 6 , 545–559 (1985)
Sikkenk, J.H., van Leeuwen, J.M.J., Sengers, J.V.: Physica 139 , 1–27 (1986)
van Leeuwen, J.M.J., Sengers, J.V.: Physica A 138 , 1–21 (1986)
Kawasaki, K., Ohta, T., Onuki, A.: Prog. Theor. Phys. (Jpn.) 63 , 821–830 (1980)
Lunacek, J.H., Cannell, D.S.: Phys. Rev. Lett. 27 , 841–844 (1971)
Calmettes, P., Laguës, I., Laj, C.: Phys. Rev. Lett. 28 , 478–480 (1972)
Kagoshima, S., Ohbayashi, K., Ikushima, A.: J. Low Temp. Phys. 11 , 765–774 (1973)
Warkulwitz, V.P., Mozer, B., Green, M.S.: Phys. Rev. Lett. 32 , 1410–1413 (1974)
Anisimov, M.A., Evtyushenkov, A.M., Kiyachenko, Yu.F., Yudin, I.K.: ZhETF Pis. Red. 20 , 378–382 (1974) [English translation: JETP Lett. 20 , 170–171 (1974)]
Lin, J.S., Schmidt, P.W.: Phys. Rev. A 10 , 2290–2305 (1974)
Dale, H., Lin, J.S., Dolesji, D.A., Casteel, J.L., Pringle, O.A., Schmidt, P.W.: Phys. Rev. A 15 , 2513–2518 (1977)
Gürmen, E., Chandrasekhar, M., Chumbley, P.E., Bale, H.D., Dolesji, D.A., Lin, J.S., Schmidt, P.W.: Phys. Rev. A 22 , 170–176 (1980)
Schneider, R., Belkoura, L., Schelten, J., Woermann, D., Chu, B.: Phys. Rev. B 22 , 5507–5516 (1980)
Schwahn, D., Belkoura, L., Woermann, D.: Ber. Bunsenges. Phys. Chem. 90 , 339–342 (1986)
Izumi, Y.: Phys. Rev. A 39 , 5826–5831 (1989)
Damay, P., Leclercq, F., Chieux, P.: Physica B 156–157 , 223–225 (1989)
Damay, P., Leclercq, F., Chieux, P.: Phys. Rev. B 40 , 4696–4708 (1989)
Bonetti, M., Calmettes, P.: Int. J. Thermophys. 19 , 1555–1566 (1998)
Tracy, C.A., McCoy, B.M.: Phys. Rev. B 12 , 368–387 (1975)
Goldburg, W.I.: In: Cummins, H.Z., Levanyuk, A.P. (eds.) Light Scattering Near Phase Transitions, pp. 531–581. North Holland, Amsterdam (1983)
Gansen, P., Janssen, T., Schön, W., Woermann, D.: Ber. Bunsenges. Phys. Chem. 84 , 1149–1156 (1980)
Shanks, J.G.: A light-scattering study of static critical phenomena in binary liquid mixtures. Ph.D. Thesis, Department of Physics and Astronomy, University of Maryland, College Park, MD (1986)
Burstyn, H.C.: An examination of the structure factor, near the consolute point, in the binary fluid 3-methylpentane + nitroethane. Ph.D. Thesis, Department of Physics and Astronomy, University of Maryland, College Park, MD (1979)
Greer, S.C.: Ber. Bunsenges. Phys. Chem. 81 , 1079–1081 (1977)
Greer, S.C.: J. Chem. Eng. Data 31 , 272–273 (1986)
Morrison, G., Knobler, C.M.: J. Chem. Phys. 65 , 5507–5517 (1976)
Hamano, K., Teshigawara, S., Koyama, T., Kuwahara, N.: Phys. Rev. A 33 , 485–489 (1986)
Puglieli, V.G., Ford, N.C.: Phys. Rev. Lett. 25 , 143–147 (1970)
Shanks, J.G., Sengers, J.V.: Phys. Rev. A 38 , 885–896 (1988)
Frisken, B.J., Feri, F., Cannell, D.S.: Phys. Rev. Lett. 66 , 2754–2757 (1991)
Bailey, A.E., Cannell, D.S.: Phys. Rev. E 50 , 4853–4864 (1994)
Bourgou, A., Beysens, D.: Phys. Rev. Lett. 47 , 257–260 (1981)
Zalczer, G., Beysens, D.: J. Chem. Phys. 92 , 6747–6754 (1990)
Hernández, M.P., Ortega, F., Rubio, R.G.: J. Chem. Phys. 119 , 4428–4436 (2003)
Gutkowski, K., Anisimov, M.A., Sengers, J.V.: J. Chem. Phys. 114 , 3133–3148 (2001)
Debye, P.: In: Green, M.S., Sengers, J.V. (eds.) Proceedings of a Conference Held in Washington, D.C., April 1965. National Bureau of Standards Miscellaneous Publ., vol. 273, p. 130. U.S. Government Printing Office, Washington (1966)
Kouvel, J.S., Fisher, M.E.: Phys. Rev. A 136 , 1626–1632 (1964)
Anisimov, M.A., Kiselev, S.B., Sengers, J.V., Tang, S.: Physica A 188 , 487–525 (1992)
Anisimov, M.A., Povodyrev, A.A., Kulikov, V.D., Sengers, J.V.: Phys. Rev. Lett. 75 , 3146–3149 (1995)
Albright, P.C., Chen, Z.Y., Sengers, J.V.: Phys. Rev. B 36 , 877–880 (1987)
Chen, Z.Y., Albright, P.C., Sengers, J.V.: Phys. Rev. A 41 , 3161–3177 (1990)
Tang, S., Sengers, J.V., Chen, Z.Y.: Physica A 179 , 344–377 (1991)
Zinn-Justin, J.: Quantum Field Theory and Critical Phenomena. Oxford University Press, Oxford (1996)
Nicoll, J.F.: Phys. Rev. A 24 , 2203–2220 (1981)
Nicoll, J.F., Bhattacharjee, J.K.: Phys. Rev. B 23 , 389–401 (1981)
Nicoll, J.F., Albright, P.C.: Phys. Rev. B 31 , 4576–4589 (1985)
Anisimov, M.A., Sengers, J.V.: In: Sengers, J.V., Kayser, R.F., Peters, C.J., White, H.J. Jr. (eds.) Equations of State for Fluids and Fluid Mixtures, pp. 381–434. Elsevier, Amsterdam (2000)
Agayan, V.A., Anisimov, M.A., Sengers, J.V.: Phys. Rev. E 64 , 026125 (2001)
Kim, Y.C., Anisimov, M.A., Sengers, J.V., Luijten, E.: J. Stat. Phys. 110 , 591–609 (2003)
Narayanan, T., Pitzer, K.S.: J. Chem. Phys. 102 , 8118–8131 (1995)
Melnichenko, Y.B., Anisimov, M.A., Povodyrev, A.A., Wignall, G.D., Sengers, J.V., Van Hook, W.A.: Phys. Rev. Lett. 79 , 5266–5269 (1997)
Weingärtner, H., Kleimeier, M., Wiegand, S., Schröer, W.: J. Stat. Phys. 78 , 169 (1995)
Weingärtner, H., Schröer, W.: Adv. Chem. Phys. 116 , 1–66 (2001)
Download references
Author information
Authors and affiliations.
Institute for Physical Science and Technology, University of Maryland, College Park, MD, 20742-8510, USA
Jan V. Sengers
Photon Research Associates/Raytheon, 9985 Pacific Heights Blvd., San Diego, CA, 92121-1723, USA
Joseph G. Shanks
You can also search for this author in PubMed Google Scholar
Corresponding author
Correspondence to Jan V. Sengers .
Rights and permissions
Reprints and permissions
About this article
Sengers, J.V., Shanks, J.G. Experimental Critical-Exponent Values for Fluids. J Stat Phys 137 , 857–877 (2009). https://doi.org/10.1007/s10955-009-9840-z
Download citation
Received : 21 June 2009
Accepted : 22 September 2009
Published : 08 October 2009
Issue Date : December 2009
DOI : https://doi.org/10.1007/s10955-009-9840-z
Share this article
Anyone you share the following link with will be able to read this content:
Sorry, a shareable link is not currently available for this article.
Provided by the Springer Nature SharedIt content-sharing initiative
- Critical correlation function
- Critical exponents
- Gravity effects
- Isobutyric acid + water
- Light scattering
- Find a journal
- Publish with us
- Track your research

An official website of the United States government
The .gov means it’s official. Federal government websites often end in .gov or .mil. Before sharing sensitive information, make sure you’re on a federal government site.
The site is secure. The https:// ensures that you are connecting to the official website and that any information you provide is encrypted and transmitted securely.
- Publications
- Account settings
- My Bibliography
- Collections
- Citation manager
Save citation to file
Email citation, add to collections.
- Create a new collection
- Add to an existing collection
Add to My Bibliography
Your saved search, create a file for external citation management software, your rss feed.
- Search in PubMed
- Search in NLM Catalog
- Add to Search
Universal window for two-dimensional critical exponents
Affiliation.
- 1 Department of Chemistry, University College London, 20 Gordon Street, London WC1H 0AJ, UK.
- PMID: 21694394
- DOI: 10.1088/0953-8984/20/27/275233
Two-dimensional condensed matter is realized in increasingly diverse forms that are accessible to experiment and of potential technological value. The properties of these systems are influenced by many length scales and reflect both generic physics and chemical detail. To unify their physical description is therefore a complex and important challenge. Here we investigate the distribution of experimentally estimated critical exponents, β, that characterize the evolution of the order parameter through the ordering transition. The distribution is found to be bimodal and bounded within a window ∼0.1≤β≤0.25, facts that are only in partial agreement with the established theory of critical phenomena. In particular, the bounded nature of the distribution is impossible to reconcile with the existing theory for one of the major universality classes of two-dimensional behaviour-the XY model with four-fold crystal field-which predicts a spectrum of non-universal exponents bounded only from below. Through a combination of numerical and renormalization group arguments we resolve the contradiction between theory and experiment and demonstrate how the 'universal window' for critical exponents observed in experiment arises from a competition between marginal operators.
PubMed Disclaimer
Similar articles
- Proceedings of the Second Workshop on Theory meets Industry (Erwin-Schrödinger-Institute (ESI), Vienna, Austria, 12-14 June 2007). Hafner J. Hafner J. J Phys Condens Matter. 2008 Feb 13;20(6):060301. doi: 10.1088/0953-8984/20/06/060301. Epub 2008 Jan 24. J Phys Condens Matter. 2008. PMID: 21693862
- Oxide surfaces. Willmott P. Willmott P. J Phys Condens Matter. 2008 Jul 2;20(26):260301. doi: 10.1088/0953-8984/20/26/260301. Epub 2008 Jun 9. J Phys Condens Matter. 2008. PMID: 21694334
- Universal amplitude ratios of the renormalization group: two-dimensional tricritical Ising model. Fioravanti D, Mussardo G, Simon P. Fioravanti D, et al. Phys Rev E Stat Nonlin Soft Matter Phys. 2001 Jan;63(1 Pt 2):016103. doi: 10.1103/PhysRevE.63.016103. Epub 2000 Dec 18. Phys Rev E Stat Nonlin Soft Matter Phys. 2001. PMID: 11304310
- Phase transition in the two-dimensional dipolar planar rotator model. Mól LA, Costa BV. Mól LA, et al. J Phys Condens Matter. 2010 Feb 3;22(4):046005. doi: 10.1088/0953-8984/22/4/046005. Epub 2010 Jan 12. J Phys Condens Matter. 2010. PMID: 21386329
- Self-avoiding walk, spin systems and renormalization. Slade G. Slade G. Proc Math Phys Eng Sci. 2019 Jan;475(2221):20180549. doi: 10.1098/rspa.2018.0549. Epub 2019 Jan 23. Proc Math Phys Eng Sci. 2019. PMID: 30760959 Free PMC article. Review.
- Competing antiferromagnetic-ferromagnetic states in a d 7 Kitaev honeycomb magnet. Vivanco HK, Trump BA, Brown CM, McQueen TM. Vivanco HK, et al. Phys Rev B. 2020 Dec;102(22):10.1103/PhysRevB.102.224411. doi: 10.1103/PhysRevB.102.224411. Phys Rev B. 2020. PMID: 37719682 Free PMC article.
- Continuous symmetry breaking in a two-dimensional Rydberg array. Chen C, Bornet G, Bintz M, Emperauger G, Leclerc L, Liu VS, Scholl P, Barredo D, Hauschild J, Chatterjee S, Schuler M, Läuchli AM, Zaletel MP, Lahaye T, Yao NY, Browaeys A. Chen C, et al. Nature. 2023 Apr;616(7958):691-695. doi: 10.1038/s41586-023-05859-2. Epub 2023 Feb 27. Nature. 2023. PMID: 36848931
- Uncovering the Kagome Ferromagnet within a Family of Metal-Organic Frameworks. Ivko SA, Tustain K, Dolling T, Abdeldaim A, Mustonen OHJ, Manuel P, Wang C, Luetkens H, Clark L. Ivko SA, et al. Chem Mater. 2022 Jun 28;34(12):5409-5421. doi: 10.1021/acs.chemmater.2c00289. Epub 2022 Jun 9. Chem Mater. 2022. PMID: 36160701 Free PMC article.
- Dynamic magnetic crossover at the origin of the hidden-order in van der Waals antiferromagnet CrSBr. López-Paz SA, Guguchia Z, Pomjakushin VY, Witteveen C, Cervellino A, Luetkens H, Casati N, Morpurgo AF, von Rohr FO. López-Paz SA, et al. Nat Commun. 2022 Aug 12;13(1):4745. doi: 10.1038/s41467-022-32290-4. Nat Commun. 2022. PMID: 35961970 Free PMC article.
- The Magnetic Genome of Two-Dimensional van der Waals Materials. Wang QH, Bedoya-Pinto A, Blei M, Dismukes AH, Hamo A, Jenkins S, Koperski M, Liu Y, Sun QC, Telford EJ, Kim HH, Augustin M, Vool U, Yin JX, Li LH, Falin A, Dean CR, Casanova F, Evans RFL, Chshiev M, Mishchenko A, Petrovic C, He R, Zhao L, Tsen AW, Gerardot BD, Brotons-Gisbert M, Guguchia Z, Roy X, Tongay S, Wang Z, Hasan MZ, Wrachtrup J, Yacoby A, Fert A, Parkin S, Novoselov KS, Dai P, Balicas L, Santos EJG. Wang QH, et al. ACS Nano. 2022 May 24;16(5):6960-7079. doi: 10.1021/acsnano.1c09150. Epub 2022 Apr 20. ACS Nano. 2022. PMID: 35442017 Free PMC article. Review.
LinkOut - more resources
Full text sources.
- IOP Publishing Ltd.
- Citation Manager
NCBI Literature Resources
MeSH PMC Bookshelf Disclaimer
The PubMed wordmark and PubMed logo are registered trademarks of the U.S. Department of Health and Human Services (HHS). Unauthorized use of these marks is strictly prohibited.

An official website of the United States government
Here’s how you know
Official websites use .gov A .gov website belongs to an official government organization in the United States.
Secure .gov websites use HTTPS A lock ( Lock A locked padlock ) or https:// means you’ve safely connected to the .gov website. Share sensitive information only on official, secure websites.
https://www.nist.gov/publications/ising-critical-exponents-real-fluids-experiment
Ising critical exponents in real fluids: An experiment
Download paper, additional citation formats.
- Google Scholar
If you have any questions about this publication or are having problems accessing it, please contact [email protected] .
- Hydrodynamics
Experimental Critical-Exponent Values for Fluids
- December 2009
- Journal of Statistical Physics 137(5):857-877
- 137(5):857-877
- This person is not on ResearchGate, or hasn't claimed this research yet.
Discover the world's research
- 25+ million members
- 160+ million publication pages
- 2.3+ billion citations
Scaling behavior of the quantum phase transition from a quantum-anomalous-Hall insulator to an axion insulator
- Xinyu Wu ORCID: orcid.org/0000-0001-9654-3285 1 ,
- Di Xiao ORCID: orcid.org/0000-0002-2894-034X 2 ,
- Chui-Zhen Chen 3 ,
- Jian Sun ORCID: orcid.org/0000-0002-4814-1676 1 ,
- Ling Zhang 2 ,
- Moses H. W. Chan 2 ,
- Nitin Samarth 2 ,
- X. C. Xie 1 , 4 , 5 ,
- Xi Lin ORCID: orcid.org/0000-0003-3383-2017 1 , 4 , 5 &
- Cui-Zu Chang ORCID: orcid.org/0000-0003-3515-2955 2
Nature Communications volume 11 , Article number: 4532 ( 2020 ) Cite this article
- Phase transitions and critical phenomena
- Quantum Hall
- Topological insulators
The phase transitions from one plateau to the next plateau or to an insulator in quantum Hall and quantum anomalous Hall (QAH) systems have revealed universal scaling behaviors. A magnetic-field-driven quantum phase transition from a QAH insulator to an axion insulator was recently demonstrated in magnetic topological insulator sandwich samples. Here, we show that the temperature dependence of the derivative of the longitudinal resistance on magnetic field at the transition point follows a characteristic power-law that indicates a universal scaling behavior for the QAH to axion insulator phase transition. Similar to the quantum Hall plateau to plateau transition, the QAH to axion insulator transition can also be understood by the Chalker–Coddington network model. We extract a critical exponent κ ~ 0.38 ± 0.02 in agreement with recent high-precision numerical results on the correlation length exponent of the Chalker–Coddington model at ν ~ 2.6, rather than the generally-accepted value of 2.33.
Similar content being viewed by others
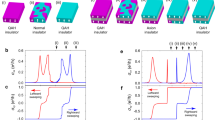
Quantized resistance revealed at the criticality of the quantum anomalous Hall phase transitions
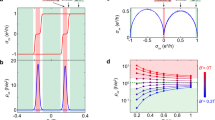
Probing the mesoscopic size limit of quantum anomalous Hall insulators
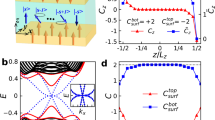
High spin axion insulator
Introduction.
The study of quantum phase transitions is a rich topic of research in condensed matter physics. Quantum phase transitions, such as the plateau-to-plateau transition in the quantum Hall (QH) effect at high magnetic field, can be accessed by varying only one physical parameter (e.g., magnetic field) near absolute zero temperature 1 . The fundamental physics of quantum phase transition can be revealed by investigating its scaling behaviors at the crossing point of magnetoresistance at different temperatures 2 , 3 , 4 , 5 . The universal scaling behavior associated with such a phase transition is usually studied by characterizing a temperature-dependent resistivity/conductivity that is governed by a single exponent κ 2 , 3 , 4 , 5 . A similar feature of crossing magnetoresistance at different temperatures has also been observed in the QH to Anderson or Hall insulator transition 6 , 7 , 8 , 9 , 10 .
The quantum anomalous Hall (QAH) state, a zero magnetic field manifestation of the integer QH state, is also a topological phase of quantum matter 11 , 12 , 13 . Similar to the QH effect 14 , the QAH effect harbors dissipationless chiral edge states with quantized Hall resistance and vanishing longitudinal resistance 15 . These edge states are spin-polarized and their chirality is determined by the internal magnetization of the sample. The QAH effect was envisioned by Duncan Haldane 11 and was first realized in magnetically doped topological insulator (TI) thin films 13 , 16 . To date, the QAH effect at zero magnetic field has been realized in the Cr- and/or V-doped TI systems 13 , 16 , 17 . An axion insulator state was recently realized in V-doped TI/TI/Cr-doped TI sandwich structures 18 , 19 . When the external magnetic field is swept between the coercive fields of the two magnetic TI films, i.e., the magnetizations of the two surfaces are antiparallel, the axion insulator state appears. The axion insulator is characterized by zero Hall resistance R xy and a very large longitudinal resistance R xx 18 , 19 . When the magnetizations of the two magnetic TI layers are driven by an external magnetic field from the antiparallel to parallel alignment, a quantum phase transition from an axion insulator to a QAH insulator has been demonstrated 18 , 19 .
In this study, we studied the scaling behavior of the quantum phase transition from the QAH insulator to the axion insulator in magnetic TI sandwich samples in the temperature range between 45 and 100 mK. We found the temperature dependence of the derivative of the longitudinal resistance R xx on the magnetic field B evaluated at the critical field B c follows a characteristic power-law behavior, i.e. \((\frac{{dR_{xx}}}{{dB}})_{B = B_{\mathrm{{c}}}} \propto T^{ - \kappa }\) . The exponent κ is found to be 0.38 ± 0.02, smaller than the previously believed value κ ∼ 0.43 for the QH plateau-to-plateau transition 4 , 20 , 21 , but in good agreement with the recent high-precision numerical results on the Chalker–Coddington network model, which yields a correlation length exponent ν ~ 2.6 if the dynamic exponent p = 2 22 , 23 , 24 , 25 . The experimental results are supported by theoretical arguments that the QAH to axion insulator phase transition, just like the QH plateau-to-plateau transition, can also be described using the Chalker–Coddington network model. In addition, by using a quasi-DC measurement circuit, we measured the two-terminal resistance R 12 in the axion insulator regime and found it to be as high as 5.0 × 10 4 h/e 2 (~1.3 GΩ) (Supplementary Note 1 ). This value suggests the highly insulating property of the topology-induced axion insulator state. By analyzing the temperature dependence of R 12 , we probed two different insulating behaviors in two adjacent temperature ranges, validating the reliability of our scaling study for the QAH to axion insulator transition.
Sample structures and sample characterizations
The heterostructure samples used in this work are sandwiches with an undoped TI layer (5QL (Bi, Sb) 2 Te 3 layer) inserted between two magnetic TI layers with a 3QL Cr-doped (Bi, Sb) 2 Te 3 layer in the bottom and a 3QL V-doped (Bi, Sb) 2 Te 3 layer on top (Fig. 1a ). Specifically, the samples are 3QL (Bi, Sb) 1.89 V 0.11 Te 3 /5QL (Bi, Sb) 2 Te 3 /3QL (Bi, Sb) 1.85 Cr 0.15 Te 3 sandwich heterostructures. These samples were grown on 0.5 mm-thick heat-treated SrTiO 3 (111) substrate in a molecular beam epitaxy (MBE) chamber with a base vacuum of 2 × 10 −10 mbar. The Bi/Sb ratio in each layer was optimized to tune the chemical potential near the charge neutral point. The transport studies were performed in two dilution refrigerators (Leiden 6 mK/14 T and 8 mK/9 T) with the magnetic field B applied perpendicular to the film plane. We used an excitation current of no more than 0.3 nA with a lowest trusted electron temperature ~45 mK (Supplementary Note 2 ). Six-terminal Hall bars with a bottom-gate electrode (Fig. 1b ) were used for electrical transport studies.
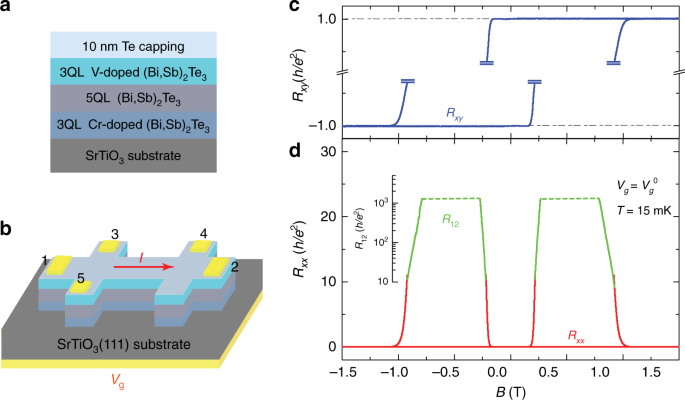
a Schematic of the magnetic/nonmagnetic/magnetic TI sandwich heterostructure. b Schematic of the Hall bar device used in electrical transport measurements. c Magnetic field B dependence of R xy (blue) at V g = V g 0 = +21.5 V. d B dependence of R xx (red) and R 12 (green) at V g = V g 0 = +21.5 V. The four-terminal longitudinal resistance R xx data are shown in red, whereas the two-terminal resistance R 12 data are shown in green. When the R 12 value is comparable with or higher than the impedance of parasitic capacitance of the measurement circuit, the R 12 values measured with AC two-terminal method are not reliable and are represented by the green dashed lines. R xy values in the highly insulating regime due to the inaccuracy caused by mutual mixing from huge R xx are not shown. Measurements in c and d were taken when the environmental temperature of the dilution fridge is 15 mK.
Figures 1c , d show the magnetic field B dependence of the Hall resistance R xy (blue) and the longitudinal resistance R xx (red) of the magnetic TI sandwich sample at T = 15 mK and V g = V g 0 = +21.5 V. Here, V g 0 is the charge neutral point, determined by achieving the largest two-terminal resistance in the axion insulator regime. When the magnetization of the bottom Cr-doped TI layer is aligned in parallel with that of the top V-doped TI layer, the sample displays a perfect QAH state: at B = 0 T, R xy (0) = ± h/e 2 (within 0.07% of quantization) and R xx (0) < 17 Ω. When the magnetization of the two magnetic TI layers is antiparallel, i.e., B c1 < B < B c2 , where B c1 and B c2 are the coercive fields of Cr-doped and V-doped TI layers, the sample shows an axion insulator state 19 . As the axion insulator is a topology-induced insulating state, its R xx value diverges. The traditional four-terminal measurement method is not suitable to measure such a large resistance. The two-terminal method with an AC signal source could deal with larger resistance but the largest resistance is still limited due to the unavoidable parasitic capacitance in the measurement circuit. Neither the traditional four-terminal measurement method nor the two-terminal method with an AC signal source provides reliable resistance values for such an insulating state. Therefore, we show four-terminal data only between 0 and ~10 h/e 2 , and AC two-terminal data from ~10 h/e 2 to ~1000 h/e 2 in Fig. 1c . At higher resistance range, we used a quasi-DC measurement scheme. This quasi-DC measurement method can yield reliable two-terminal resistance up to ~2 GΩ (~7.7 × 10 4 h/e 2 ) (Supplementary Note 1 ). This method, however, is inconvenient for measurement when sweeping the magnetic field, because it requires very long measurement time and does not provide a “continuous” field-dependent resistance value as the AC lock-in technique.
QAH insulator to axion insulator quantum phase transition
When B > B c2 > B c1 , the sample shows the perfect QAH state (Fig. 1c ), so there must be a magnetic-field-driven quantum phase transition from an axion insulator to a QAH insulator near B c2 . To study the scaling behavior of this quantum phase transition, we measured the magnetic field B dependence of R xx around B c2 at different temperatures, as shown in Fig. 2a . All R xx − B curves in the temperature range 45 mK ≤ T ≤ 80 mK cross each other at one point, specifically at the critical magnetic field B = B c ~ 0.979 T. B c separates the R xx − B curves into two regions. For B < B c , R xx increases with lowering temperature, showing an “insulating” behavior. This “insulating” state corresponds to the axion insulator state with a large R xx and zero R xy near-zero temperature 18 , 19 . For B > B c , R xx decreases with lowering temperature, exhibiting a “metallic” behavior. This “metallic” state corresponds to the chiral edge channel of the QAH insulator state with zero R xx and quantized R xy at zero temperature 13 , 16 . This “metal” to insulator quantum phase transition is further demonstrated from the temperature dependence of R xx measured at various magnetic fields (Fig. 2b ). Figure 2c shows the value of R xx at B = B c for different temperatures. The R xx value at T = 100 mK shows a clear deviation from the values measured at lower temperatures, which implies that we should confine our investigation of the scaling behavior of the QAH to axion insulator transition in the temperature range of 45 ≤ T ≤ 80 mK. It is noteworthy that one more transition from the QAH insulator to the axion insulator occurs at B c1 ~ 0.20 T. We experienced instability problem of our magnet at low magnetic field region, so the data acquired near B c1 cannot be used for scaling analysis.
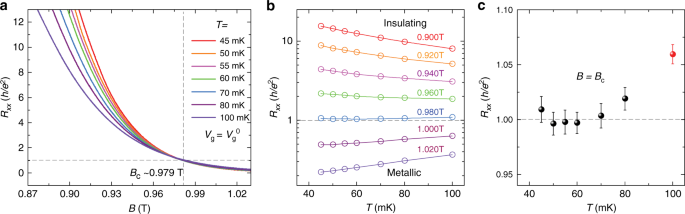
a Magnetic field dependence of R xx at various temperatures. All curves cross at one critical point at B c ~ 0.979 T. b Temperature dependence of R xx at different magnetic fields. The sample shows “insulating” behavior for B < B c and “metallic” behavior for B > B c . At B = 0.980 T, R xx is nearly independent of temperature. c R xx value at the crossing point as a function of temperature.

Scaling analysis of the QAH insulator to axion insulator transition
Next, we analyze the scaling behavior of the QAH to axion insulator phase transition. We follow the scaling analysis of the QH plateau-to-plateau transition 2 , 3 and scale the B dependence of R xx at different temperatures to a single parameter relation: \((\frac{{dR_{xx}}}{{dB}})_{B = B_{\mathrm{{c}}}} \propto T^{ - \kappa }\) . Figure 3a shows the \((\frac{{dR_{xx}}}{{dB}})_{B = B_{\mathrm{{c}}}}\) at different temperatures plotted on log–log scale. The perfect linear fit in the temperature range 45 mK ≤ T ≤ 80 mK demonstrates the QAH to axion insulator quantum phase transition does show the universal scaling behavior. For T < 45 mK, \((\frac{{dR_{xx}}}{{dB}})_{B = B_{\mathrm{{c}}}}\) starts to deviate from the linear dependence, leading us to suspect that the real electron temperature of the sample is higher than the value registered by the thermometer on the mixing chamber in this temperature range (Supplementary Note 2 ). We note that κ can also be acquired in the R xy analysis over the same temperature range, but it has a lower accuracy due to mutual mixing from huge R xx (Supplementary Note 3 ).
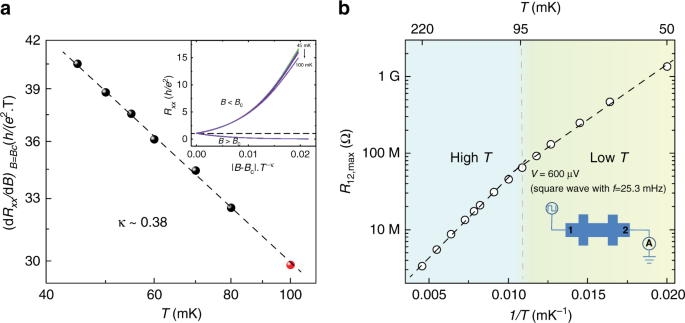
a Temperature dependence of \((\frac{{dR_{xx}}}{{dB}})_{B = B_{\mathrm{{c}}}}\) on log–log scale. The black dashed line is the linear fit. The inset shows R xx as a function of the s c aled magnetic field | B − B c |· T −κ at different temperatures. b The two-terminal DC resistance R 12 as a function of 1/ T (bottom axis) or T (top axis) when the sample shows the most insulating state (i.e., B = 0.40 T). The vertical axis is on a log scale. The inset shows the measurement circuit we used to accurately measure the huge R 12 of the axion insulator state.
From the linear fit in Fig. 3a , we extracted a critical exponent κ ~ 0.38 ± 0.02. To further demonstrate the universal scaling behavior of the QAH to axion insulator transition, we used the one-parameter scaling relation \(R_{xx} = f[\left| {B - B_{\mathrm{c}}} \right| \cdot T^{ - \kappa }]\) to scale the B dependence of R xx at different temperatures as a function of scaled magnetic field \(\left| {B - B_{\mathrm{c}}} \right| \cdot T^{ - \kappa }\) 26 , 27 . All curves except the curve at T = 100 mK are well-scaled to a single curve around the critical point. Figure 3a shows that including the 100 mK data for the scaling fit does not change κ , but if more high-temperature data points are included, a larger κ is found but not reliable (Supplementary Note 4 ). Below, we propose a theoretical picture to explain why the QAH to the axion insulator transition shares the same universality class of QH plateau-to-plateau transition and also demonstrate that κ ~ 0.38 ± 0.02 is in agreement with the latest high-precision numerical results of the correlation length exponent ν ~ 2.6 22 , 23 , 24 , 25 .
Theoretical analysis of the QAH insulator to axion insulator transition
The top and bottom surface states in V-doped TI/TI/Cr-doped TI sandwich heterostructures can be described by the effective Hamiltonian:
Here, s x,y,z are Pauli matrices acting on spin space, k x,y are wave vectors in x and y directions, and v F is the Fermi velocity. The Dirac mass term M t,b and Δ t,b (r) represent spatial-averaged and random parts of the exchange field in the z -direction due to the magnetization on top and bottom surfaces, respectively, where “ t ” and “ b ” denote the top and bottom surfaces. The random scalar potential V t,b ( r ) denotes an inhomogeneous onsite potential inside the sandwich sample. In the clean limit, V t,b ( r ) = 0 and Δ t,b ( r ) = 0, so the Hall conductance of the top and bottom surfaces \({\upsigma}_{xy}^{{\mathrm{t}},{\mathrm{b}}} = {\mathrm{sign}}(M_{{\mathrm{t}},{\mathrm{b}}})e^2/2{\mathrm{h}}\) is determined by the sign of magnetization sign( M t,b ). The QAH phase with \({\upsigma}_{xy}^{\mathrm{t}} = {\upsigma}_{xy}^{\mathrm{b}}\) and the axion insulator phase with \({\upsigma}_{xy}^{\mathrm{t}} = - {\upsigma}_{xy}^{\mathrm{b}}\) require parallel and antiparallel magnetizations on the top and bottom surfaces, respectively. During the magnetization reversal process, the magnetization of one surface switches direction and a magnetic-field-driven quantum phase transition from the QAH insulator to the axion insulator occurs. Therefore, we consider only one surface in the following and denote it as top surface for concreteness. At the critical point of the QAH insulator to the axion insulator transition, the spatial-averaged magnetization of the top surface M t = 0, whereas \({\Delta}_{\mathrm{t}}\left( {{r}} \right) \ne 0\) with an equal population of upward and downward magnetic domains (Fig. 4a ). Now, the top surface state can be described by the random Dirac Hamiltonian with randomness in the mass Δ t ( r ) and the scalar potential V t ( r ):
There exists a chiral edge mode between two regions with opposite masses for the Dirac Hamiltonian 28 . Due to a spatially varying random Dirac mass Δ t ( r ), the random Dirac Hamiltonian H t exhibits chiral edge modes confined to the zero-mass contours Δ t ( r ) = 0 (Fig. 4a ) 28 .
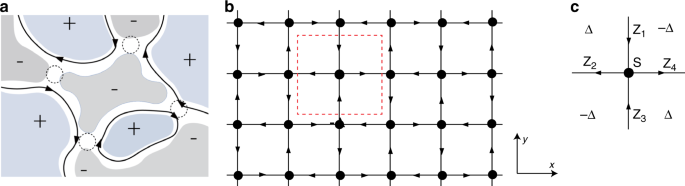
a The chiral zero-energy edge states along contours of zero-mass domain walls of Dirac fermions. “ + ” and “−” denote domains of positive and negative masses of Dirac fermions, respectively. b The Chalker–Coddington network model on a square lattice. c At each node (red dashed square in b ), a scattering matrix S describes the scattering from incoming (labeled by Z i = 1,3 ) to outgoing modes (labeled by Z i = 2,4 ). The chiral edge modes can be created by Dirac Hamiltonian with four domains of opposite Dirac masses labeled by ±Δ .
Moreover, it is known that the Chalker–Coddington network model can be mapped to the random Dirac Hamiltonian 28 , 29 , 30 . Figure 4b shows the Chalker–Coddington network model on a square lattice structure. At each node (red dashed square in Fig. 4b ), a scattering matrix S describes the scattering from incoming (labeled by Z i = 1,3 ) to outgoing channels (labeled by Z i = 2,4 ) (Fig. 4c ). Here, the incoming and outgoing channels in the network model correspond to the chiral edge modes at zero-mass domain walls of the random Dirac Hamiltonian (Fig. 4a ). Figure 4c shows the chiral edge channels at the four domains of opposite masses (labeled by ±Δ t ). All chiral edge channels in the network model can be created similarly and the Chalker–Coddington model is thus equivalent to the random Dirac Hamiltonian 28 , 30 . As the QH plateau-to-plateau transition (without electron–electron interactions) can also be described by the Chalker–Coddington model 29 , the quantum phase transition from the QAH insulator to the axion insulator must share the same universality class of QH plateau-to-plateau transition. Indeed, the critical exponent κ ~ 0.38 ± 0.02 revealed in our experiment is close to the generally accepted value κ ∼ 0.43 in the QH plateau-to-plateau transition 4 , 20 , 21 . The slight discrepancy of κ = p /2 ν may result from a decreased dynamic exponent p or an increased correlation exponent ν . However, the theoretical problem of the QH plateau-to-plateau transition with strong electron–electron interactions remains open and a reliable method for calculating these exponents is yet to be established 31 , 32 , 33 , 34 , 35 , 36 . Recently, the high-precision numerical calculations show that the correlation length exponent ν of the Chalker–Coddington model is ~2.6 22 , 23 , 24 instead of ~2.33. It is noteworthy that the larger ν -value is also supported by the recent field theory analysis 25 . As a consequence, the critical exponent κ = p /2 ν ∼ 0.38, if we assume p = 2 is in good agreement with the κ- value obtained in our experiment. Here, the exponent p = 2, exceeding the non-interacting value p = 1, may be induced by the appearance of the Coulomb interaction, similar to the discussion for the QH plateau-to-plateau transition 31 , 32 , 33 , 34 , 35 , 36 . In our samples, as the density of states of Dirac fermions is low, the long-range Coulomb interaction is unscreened and the triplet interaction channel is eliminated due to the strong spin-orbital coupling of TI materials 32 . We note that the QAH to axion insulator transition in magnetic TI sandwiches belongs to the QH-type instead of the Berezinskii–Kosterlitz–Thouless-type transition as we predicted in an individual magnetic TI thin film with random domains 37 . In such an individual magnetic TI thin film, the top and bottom surfaces are coupled and play a joint role, so this case is described by the Chalker–Coddington model with two channels 37 , 38 . In other words, the magnetization reversal during the QAH plateau-to-plateau transition occurs on both the top and bottom surfaces. This may be the reason why a different κ ~ 0.6 was found for the QAH to Anderson insulator transition 39 and the QAH plateau-to-plateau transition 40 . However, the magnetization reversal in magnetic TI sandwich heterostructures during the QAH insulator to the axion insulator transition occurs on only one surface.
A recent study on the quantum phase transition between a Chern insulator to an axion insulator driven by the external magnetic field in even number layers of topological antiferromagnet MnBi 2 Te 4 reports a critical exponent κ ~ 0.47 41 . In the QH plateau-to-plateau transition, values of κ ranging from 0.15 to ~0.8 have been reported in the two-dimensional electron/hole gas samples 2 , 5 , 42 , 43 , 44 , 45 , 46 , 47 , 48 , 49 . Several factors can cause larger κ , e.g., the high concentration of ionized impurities or clustered impurities 4 . If the quantum percolation is turning into a classical percolation, κ is expected to eventually increase to 0.75 31 , 50 . It is noteworthy that the determination of κ is also sensitive to experimental issues, e.g., the small temperature regime where all curves crossing each other as shown in Fig. 2c . It is not appropriate to include the high-temperature data in our scaling analysis, which can cause a larger and nonreliable κ (Supplementary Note 4 ). We speculate that the appropriate amount of disorder in our sample might make κ as close as to the expected value based on the recent high-precision numerical results of the correlation length exponent ν ~ 2.6 22 , 23 , 24 , 25 .
As noted above, the AC two-terminal measurement is not reliable when R 12 > 10 3 h/e 2 . To measure R 12 accurately in the most insulating regime, we employed the quasi-DC measurement method. The quasi-DC method uses a low frequency ( f = 25.3 mHz) square wave as output, which can eliminate the influence of parasitic capacitance. The thermal voltage can be removed by taking the difference of the square wave generated current. Therefore, the quasi-DC method can provide more accurate resistance values when R 12 is between ~25.8 MΩ (~ 10 3 h/e 2 ) and 2 GΩ. By varying the magnetic field B , the most insulating state R 12,max ~ 1.3 GΩ (~5.0 × 10 4 h/e 2 ) was found at B = 0.40 T at T = 50 mK (Supplementary Note 5 ). The lowest R xx in QAH state measured at 50 mK is ~20 Ω, so this suggests an order of ~10 8 ON/OFF resistance ratio in our magnetic TI sandwich samples.
Figure 3b shows the maximum two-terminal resistance R 12,max of the axion insulator state measured at different temperatures between 50 mK and 220 mK. The logarithmic R 12,max shows a linear dependence on T −1 with a slope ~333 mK below 95 mK and a steeper slope ~478 mK above 95 mK (Supplementary Note 5 ). We note that for T ≥ 100 mK, the scaling behavior starts to disappear (inset of Fig. 3a ). Therefore, the insulating behavior at the high-temperature regime might involve the contribution from the classical thermal activation, whereas the low-temperature regime reflects solely the property of an unconventional insulating quantum state (i.e., axion insulator) favoring zero-temperature limit. This further validates our choice to carry out scaling behavior study between 45 mK and 80 mK.
To summarize, we analyzed the critical scaling behavior of the quantum phase transition from a QAH insulator to an axion insulator state in magnetic TI sandwich samples. We found that the derivative of the longitudinal resistance R xx at the critical magnetic field follows a power-law dependence on temperature. Our experimental results combined with theoretical studies on the Chalker–Coddington model demonstrated that the QAH to axion insulator phase transition shares the same universality class with the QH plateau-to-plateau transition instead of the QAH plateau-to-plateau transition. Our work opens the door for further explorations of the critical behaviors of quantum phase transitions in topological materials.
Fabrications of magnetic TI sandwich Hall bar device
The magnetic TI sandwich heterostructure growth was carried out using a commercial MBE system with a vacuum ~2 × 10 −10 mbar. The heat-treated insulating SrTiO 3 (111) substrates were outgassed at ~530 °C for 1 h before the growth of the TI sandwich heterostructures. High-purity Bi (99.999%), Sb (99.9999%), Cr (99.999%), and Te (99.9999%) were evaporated from Knudsen effusion cells. During the growth of the TI, the substrate was maintained at ~240 °C. The flux ratio of Te per (Bi + Sb) was set to be >10 to prevent Te deficiency in the samples. To avoid possible contamination, a 10 nm-thick Te layer is deposited at room temperature on top of the magnetic TI sandwich heterostructures prior to their removal from the MBE chamber. Our prior study has demonstrated that the Te capping layer is much more insulating than the axion insulator in the low-temperature regime and plays a negligible effect in our electrical transport measurements 51 . We next scratched these magnetic TI heterostructure samples into a Hall bar geometry (Supplementary Note 6 ) using a computer-controlled probe station. The effective area of the Hall bar devices is ~1 mm × 0.5 mm. The electrical ohmic-contacts for dilution measurements were made by pressing indium spheres on the Hall bar. The bottom-gate electrode was prepared through an indium foil on the back side of the SrTiO 3 substrate.
Transport measurements
Transport measurements were performed using two dilution refrigerators (Leiden 6 mK/14 T and 8 mK/9 T) with the magnetic field applied perpendicular to the film plane. The bottom-gate voltage was applied using a Keithley 2400. The four-terminal measurements are obtained by applying current from 1 to 2 (Fig. 1b ), and R xx = V 34 /I 12 while R xy = V 35 /I 12 . The excitation current in the dilution refrigerator measurements is ≤0.3 nA to suppress the heating effect. The lowest trusted electron temperature in our dilution transport measurements is ~45 mK (Supplementary Note 2 ). More details about the dilution measurements can be found in the Supplementary Information .
Effective model for theoretical analysis
In the Chalker–Coddington network model, we consider a square lattice of nodes and there are two incoming and two outgoing channels at each node. Here, the wave function for a particle on each link is represented by the probability amplitudes Z i = 1,…,4 , where a random phase φ i ∈ [0, 2π] is accumulated along with the link. Because of current conservation, \(|Z_1|^2 + |Z_3|^2 = |Z_2|^2 + |Z_4|^2\) . Therefore, the two incoming channels (labeled by Z i = 1,3 ) and two outgoing channels (labeled by Z i = 2,4 ) at the node can be related by a 2 × 2 scattering matrix S
where α is a real parameter characterizing the tunneling. If α is identical for all nodes, the network model is critical at α = α c = π/4. In the experiment, the critical states are described by a random Dirac Hamiltonian with randomness in the mass Δ t ( r ) and the scalar potential V t ( r ): \(H_{\mathrm{t}} = \hbar v_{\mathrm{F}}( {s_xk_y\: - \:s_yk_x} ) + {\Delta}_{\mathrm{t}}\left( {\mathrm{r}} \right)s_z + V_t(r)\) . Here, the links in the network model are related to the chiral edge modes along contours of zero-mass domain walls, whereas the scattering parameter α at the nodes can be obtained by solving the eigenvalue equation of H t . In this Hamiltonian, s x,y,z are Pauli matrices acting on spin space, k x,y are wave vectors along x and y directions, and v F is the Fermi velocity.
Data availability
The data that support the findings of this study are available from X.L. or C.-Z. Chang upon reasonable request.
Sondhi, S. L., Girvin, S. M., Carini, J. P. & Shahar, D. Continuous quantum phase transitions. Rev. Mod. Phys. 69 , 315–333 (1997).
ADS Google Scholar
Wei, H. P., Tsui, D. C., Paalanen, M. A. & Pruisken, A. M. M. Experiments on delocalization and universality in the integral quantum Hall-effect. Phys. Rev. Lett. 61 , 1294–1296 (1988).
ADS CAS PubMed Google Scholar
Pruisken, A. M. M. Universal singularities in the integral quantum Hall-effect. Phys. Rev. Lett. 61 , 1297–1300 (1988).
Li, W. L., Csathy, G. A., Tsui, D. C., Pfeiffer, L. N. & West, K. W. Scaling and universality of integer quantum Hall plateau-to-plateau transitions. Phys. Rev. Lett. 94 , 206807 (2005).
ADS PubMed Google Scholar
Li, W. L. et al. Scaling in plateau-to-plateau transition: a direct connection of quantum Hall systems with the Anderson localization model. Phys. Rev. Lett. 102 , 216801 (2009).
Jiang, H. W., Johnson, C. E., Wang, K. L. & Hannahs, S. T. Observation of magnetic-field-induced delocalization - transition from Anderson insulator to quantum Hall conductor. Phys. Rev. Lett. 71 , 1439–1442 (1993).
Shahar, D., Tsui, D. C., Shayegan, M., Bhatt, R. N. & Cunningham, J. E. Universal conductivity at the quantum Hall liquid to insulator transition. Phys. Rev. Lett. 74 , 4511–4514 (1995).
Song, S. H., Shahar, D., Tsui, D. C., Xie, Y. H. & Monroe, D. New universality at the magnetic field driven insulator to integer quantum Hall effect transitions. Phys. Rev. Lett. 78 , 2200–2203 (1997).
ADS CAS Google Scholar
van Schaijk, R. T. F., de Visser, A., Olsthoorn, S. M., Wei, H. P. & Pruisken, A. M. M. Probing the plateau-insulator quantum phase transition in the quantum Hall regime. Phys. Rev. Lett. 84 , 1567–1570 (2000).
Smorchkova, I. P., Samarth, N., Kikkawa, J. M. & Awschalom, D. D. Giant magnetoresistance and quantum phase transitions in strongly localized magnetic two-dimensional electron gases. Phys. Rev. B 58 , R4238–R4241 (1998).
Haldane, F. D. M. Model for a quantum Hall-effect without Landau levels: condensed-matter realization of the “parity anomaly”. Phys. Rev. Lett. 61 , 2015–2018 (1988).
ADS MathSciNet CAS PubMed Google Scholar
Yu, R. et al. Quantized anomalous Hall effect in magnetic topological insulators. Science 329 , 61–64 (2010).
Chang, C. Z. et al. Experimental observation of the quantum anomalous Hall effect in a magnetic topological insulator. Science 340 , 167–170 (2013).
von Klitzing, K., Dorda, G. & Pepper, M. New method for high-accuracy determination of the fine-structure constant based on quantized Hall resistance. Phys. Rev. Lett. 45 , 494–497 (1980).
Chang, C. Z. et al. Zero-field dissipationless chiral edge transport and the nature of dissipation in the quantum anomalous Hall state. Phys. Rev. Lett. 115 , 057206 (2015).
Chang, C. Z. et al. High-precision realization of robust quantum anomalous Hall state in a hard ferromagnetic topological insulator. Nat. Mater. 14 , 473–477 (2015).
Ou, Y. et al. Enhancing the quantum anomalous Hall effect by magnetic codoping in a topological insulator. Adv. Mater. 30 , 1703062 (2017).
Google Scholar
Mogi, M. et al. Tailoring tricolor structure of magnetic topological insulator for robust axion insulator. Sci. Adv. 3 , eaao1669 (2017).
PubMed PubMed Central Google Scholar
Xiao, D. et al. Realization of the axion insulator state in quantum anomalous Hall sandwich heterostructures. Phys. Rev. Lett. 120 , 056801 (2018).
Huckestein, B. One-parameter scaling in the lowest Landau band. Phys. A 167 , 175–187 (1990).
Huckestein, B. Scaling theory of the integer quantum Hall-effect. Rev. Mod. Phys. 67 , 357–396 (1995).
Slevin, K. & Ohtsuki, T. Critical exponent for the quantum Hall transition. Phys. Rev. B 80 , 041304 (2009).
Amado, M., Malyshev, A. V., Sedrakyan, A. & Dominguez-Adame, F. Numerical study of the localization length critical index in a network model of plateau-plateau transitions in the quantum Hall effect. Phys. Rev. Lett. 107 , 066402 (2011).
Obuse, H., Gruzberg, I. A. & Evers, F. Finite-size effects and irrelevant corrections to scaling near the integer quantum Hall transition. Phys. Rev. Lett. 109 , 206804 (2012).
Zirnbauer, M. R. The integer quantum Hall plateau transition is a current algebra after all. Nucl. Phys. B 941 , 458–506 (2019).
ADS MathSciNet CAS MATH Google Scholar
Wang, T., Clark, K. P., Spencer, G. F., Mack, A. M. & Kirk, W. P. Magnetic-field-induced metal-insulator-transition in two dimensions. Phys. Rev. Lett. 72 , 709–712 (1994).
Shahar, D., Tsui, D. C., Shayegan, M., Shimshoni, E. & Sondhi, S. L. A different view of the quantum Hall plateau-to-plateau transitions. Phys. Rev. Lett. 79 , 479–482 (1997).
Ludwig, A. W. W., Fisher, M. P. A., Shankar, R. & Grinstein, G. Integer quantum Hall transition - an alternative approach and exact results. Phys. Rev. B 50 , 7526–7552 (1994).
Chalker, J. T. & Coddington, P. D. Percolation, quantum tunnelling and the integer Hall-effect. J. Phys. C. Solid State 21 , 2665–2679 (1988).
Ho, C. A. & Chalker, J. T. Models for the integer quantum hall effect: the network model, the Dirac equation, and a tight-binding Hamiltonian. Phys. Rev. B 54 , 8708–8713 (1996).
Lee, D. H., Wang, Z. Q. & Kivelson, S. Quantum percolation and plateau transitions in the quantum Hall-effect. Phys. Rev. Lett. 70 , 4130–4133 (1993).
Belitz, D. & Kirkpatrick, T. R. The Anderson-Mott Transition. Rev. Mod. Phys. 66 , 261–390 (1994).
Yang, S. R. E. & Macdonald, A. H. Coulomb gaps in a strong magnetic-field. Phys. Rev. Lett. 70 , 4110–4113 (1993).
Lee, D. H. & Wang, Z. Q. Effects of electron-electron interactions on the integer quantum Hall transitions. Phys. Rev. Lett. 76 , 4014–4017 (1996).
Wang, Z. Q., Fisher, M. P. A., Girvin, S. M. & Chalker, J. T. Short-range interactions and scaling near integer quantum Hall transitions. Phys. Rev. B 61 , 8326–8333 (2000).
Burmistrov, I. S., Bera, S., Evers, F., Gornyi, I. V. & Mirlin, A. D. Wave function multifractality and dephasing at metal-insulator and quantum Hall transitions. Ann. Phys. 326 , 1457–1478 (2011).
ADS CAS MATH Google Scholar
Chen, C. Z., Liu, H. W. & Xie, X. C. Effects of random domains on the zero Hall plateau in the quantum anomalous Hall effect. Phys. Rev. Lett. 122 , 026601 (2019).
Xiong, G., Wang, S. D., Niu, Q., Tian, D. C. & Wang, X. R. Metallic phase in quantum Hall systems due to inter-Landau-band mixing. Phys. Rev. Lett. 87 , 216802 (2001).
Chang, C. Z. et al. Observation of the quantum anomalous Hall insulator to Anderson insulator quantum phase transition and its scaling behavior. Phys. Rev. Lett. 117 , 126802 (2016).
Kawamura, M. et al. Topological quantum phase transition in magnetic topological insulator upon magnetization rotation. Phys. Rev. B 98 , 140404 (2018).
Liu, C. et al. Robust axion insulator and Chern insulator phases in a two-dimensional antiferromagnetic topological insulator. Nat. Mater. 19 , 522–527 (2020).
Wakabayashi, J., Yamane, M. & Kawaji, S. Experiments on the critical exponent of localization in Landau subbands with the Landau quantum numbers 0 and 1 in Si-Mos inversion-layers. J. Phys. Soc. Jpn. 58 , 1903–1905 (1989).
Koch, S., Haug, R. J., Vonklitzing, K. & Ploog, K. Experiments on scaling in Alxga1-Xas/Gaas heterostructures under quantum Hall conditions. Phys. Rev. B 43 , 6828–6831 (1991).
Koch, S., Haug, R. J., Vonklitzing, K. & Ploog, K. Size-dependent analysis of the metal-insulator-transition in the integral quantum Hall-effect. Phys. Rev. Lett. 67 , 883–886 (1991).
Wei, H. P., Lin, S. Y., Tsui, D. C. & Pruisken, A. M. M. Effect of long-range potential fluctuations on scaling in the integer quantum Hall-effect. Phys. Rev. B 45 , 3926–3928 (1992).
Koch, S., Haug, R. J., Vonklitzing, K. & Ploog, K. Experimental studies of the localization transition in the quantum Hall regime. Phys. Rev. B 46 , 1596–1602 (1992).
Liu, C. X. et al. Oscillatory crossover from two-dimensional to three-dimensional topological insulators. Phys. Rev. B 81 , 041307 (2010).
Wang, X. B. et al. Scaling properties of the plateau transitions in the two-dimensional hole gas system. Phys. Rev. B 93 , 075307 (2016).
Shan, P. J. et al. Coherence length saturation at the low temperature limit in two-dimensional hole gas. Phys. E 99 , 118–122 (2018).
CAS Google Scholar
Trugman, S. A. Localization, percolation, and the quantum Hall-effect. Phys. Rev. B 27 , 7539–7546 (1983).
Zhang, J. S. et al. Band structure engineering in (Bi 1-x Sb x ) 2 Te 3 ternary topological insulators. Nat. Commun. 2 , 574 (2011).
Download references
Acknowledgements
We thank C. X. Liu for helpful discussion. The work in China is supported by the NSFC (11674009), the National Key Research and Development Program of China (2017YFA0303301), NSFC (11534001 and 110801719), the Beijing Natural Science Foundation (JQ18002), the Strategic Priority Research Program of Chinese Academy of Sciences (Grant XDB28000000), and the Priority Academic Program Development of Jiangsu Higher Education Institutions and NSFC of Jiangsu province (BK20190813). C.-Z. Chang and M.H.W.C. acknowledge the support from the DOE grant (DE-SC0019064). N.S. acknowledges the support from the Penn State 2DCC-MIP under NSF grant DMR-1539916. C.-Z. Chang acknowledges the support from ARO Young Investigator Program Award (W911NF1810198) and the Gordon and Betty Moore Foundation’s EPiQS Initiative (Grant GBMF9063 to C.-Z. Chang).
Author information
Authors and affiliations.
International Center for Quantum Materials, Peking University, Beijing, 100871, China
Xinyu Wu, Jian Sun, X. C. Xie & Xi Lin
Department of Physics, The Pennsylvania State University, University Park, PA, 16802, USA
Di Xiao, Ling Zhang, Moses H. W. Chan, Nitin Samarth & Cui-Zu Chang
Institute for Advanced Study and School of Physical Science and Technology, Soochow University, Suzhou, 215006, China
Chui-Zhen Chen
Beijing Academy of Quantum Information Sciences, Beijing, 100193, China
X. C. Xie & Xi Lin
CAS Center for Excellence in Topological Quantum Computation, University of Chinese Academy of Sciences, Beijing, 100190, China
You can also search for this author in PubMed Google Scholar
Contributions
C.-Z. Chang and X.L. conceived and designed the experiment. D.X. and L.Z. grew the sandwich heterostructure samples with the help of M.H.W.C., N.S., and C.-Z. Chang. X.W. and J.S. performed the dilution refrigerator measurements with the help of L.X. C.-Z. Chen and X.C.X. provided theoretical support and did all theoretical calculations. X.W., C.-Z. Chen, X.L., and C.-Z. Chang analyzed the data and wrote the manuscript with contributions from all authors.
Corresponding authors
Correspondence to Xi Lin or Cui-Zu Chang .
Ethics declarations
Competing interests.
The authors declare no competing interests.
Additional information
Peer review information Nature Communications thanks Prosper Ngabonziza and the other, anonymous, reviewer(s) for their contribution to the peer review of this work.
Publisher’s note Springer Nature remains neutral with regard to jurisdictional claims in published maps and institutional affiliations.
Supplementary information
Supplementary information, rights and permissions.
Open Access This article is licensed under a Creative Commons Attribution 4.0 International License, which permits use, sharing, adaptation, distribution and reproduction in any medium or format, as long as you give appropriate credit to the original author(s) and the source, provide a link to the Creative Commons license, and indicate if changes were made. The images or other third party material in this article are included in the article’s Creative Commons license, unless indicated otherwise in a credit line to the material. If material is not included in the article’s Creative Commons license and your intended use is not permitted by statutory regulation or exceeds the permitted use, you will need to obtain permission directly from the copyright holder. To view a copy of this license, visit http://creativecommons.org/licenses/by/4.0/ .
Reprints and permissions
About this article
Cite this article.
Wu, X., Xiao, D., Chen, CZ. et al. Scaling behavior of the quantum phase transition from a quantum-anomalous-Hall insulator to an axion insulator. Nat Commun 11 , 4532 (2020). https://doi.org/10.1038/s41467-020-18312-z
Download citation
Received : 15 January 2020
Accepted : 18 August 2020
Published : 10 September 2020
DOI : https://doi.org/10.1038/s41467-020-18312-z
Share this article
Anyone you share the following link with will be able to read this content:
Sorry, a shareable link is not currently available for this article.
Provided by the Springer Nature SharedIt content-sharing initiative
By submitting a comment you agree to abide by our Terms and Community Guidelines . If you find something abusive or that does not comply with our terms or guidelines please flag it as inappropriate.
Quick links
- Explore articles by subject
- Guide to authors
- Editorial policies
Sign up for the Nature Briefing newsletter — what matters in science, free to your inbox daily.

An undergraduate laboratory experiment to determine the critical point of SF6 using light scattering at selected wavelengths.
- Photon Physics Group
Research output : Contribution to journal › Article › peer-review
Original language | English |
---|---|
Journal | |
Publication status | Accepted/In press - 25 Jun 2020 |
Embargoed Document
critical opal experiment EJP
Accepted author manuscript, 1.92 MB
Embargo ends: 1/01/30
Fingerprint
- Selected Wavelength Engineering 100%
- Room Temperature Engineering 100%
- Critical Point Engineering 100%
- Ambient Reaction Temperature Chemistry 100%
- Light Scattering Chemistry 100%
- Pressure Increase Engineering 50%
- Light Condition Engineering 50%
- Density Fluctuation Engineering 50%
T1 - An undergraduate laboratory experiment to determine the critical point of SF6 using light scattering at selected wavelengths.
AU - Murray, Andrew
AU - Harvey, Matthew
PY - 2020/6/25
Y1 - 2020/6/25
N2 - Molecules and atoms that are normally gaseous at room temperature and pressure will liquefy or solidify as their temperature reduces or their pressure increases. In the apparatus detailed here a commercial cell contains pressurised SF6 so that it exists in both gaseous and liquid states, with the density of both being similar at room temperature. The critical point on the phase diagram can then be investigated, by elevating the temperature of the cell so the densities of liquid and gas equalize. Under these conditions light passing through the cell is highly scattered by density fluctuations in the fluid. By measuring the transmitted light as a function of both temperature and wavelength, the critical exponent can be derived and the effects of light scattering with wavelength investigated.
AB - Molecules and atoms that are normally gaseous at room temperature and pressure will liquefy or solidify as their temperature reduces or their pressure increases. In the apparatus detailed here a commercial cell contains pressurised SF6 so that it exists in both gaseous and liquid states, with the density of both being similar at room temperature. The critical point on the phase diagram can then be investigated, by elevating the temperature of the cell so the densities of liquid and gas equalize. Under these conditions light passing through the cell is highly scattered by density fluctuations in the fluid. By measuring the transmitted light as a function of both temperature and wavelength, the critical exponent can be derived and the effects of light scattering with wavelength investigated.
M3 - Article
SN - 0143-0807
JO - European Journal of Physics
JF - European Journal of Physics
Physical Review B
Covering condensed matter and materials physics.
- Collections
- Editorial Team
- Featured in Physics
- Editors' Suggestion
- Rapid Communication
Critical exponent for the quantum Hall transition
Keith slevin and tomi ohtsuki, phys. rev. b 80 , 041304(r) – published 9 july 2009, see synopsis: a coincidence of errors.
- Citing Articles (122)
- ACKNOWLEDGEMENTS
We report an estimate ν = 2.593 [2.587,2.598] of the critical exponent of the Chalker-Coddington model of the integer quantum Hall effect that is significantly larger than previous numerical estimates and in disagreement with experiment. This suggests that models of noninteracting electrons cannot explain the critical phenomena of the integer quantum Hall effect.
- Received 13 May 2009
DOI: https://doi.org/10.1103/PhysRevB.80.041304
©2009 American Physical Society
A coincidence of errors
Published 17 august 2009.
In integer quantum Hall systems, do theory and experiment agree for the wrong reasons?
See more in Physics
Authors & Affiliations
- Department of Physics, Graduate School of Science, Osaka University, 1-1 Machikaneyama, Toyonaka, Osaka 560-0043, Japan
- Department of Physics, Sophia University, Kioi-cho 7-1, Chiyoda-ku, Tokyo 102-8554, Japan
Article Text (Subscription Required)
References (subscription required).
Vol. 80, Iss. 4 — 15 July 2009
Access Options
- Buy Article »
- Log in with individual APS Journal Account »
- Log in with a username/password provided by your institution »
- Get access through a U.S. public or high school library »
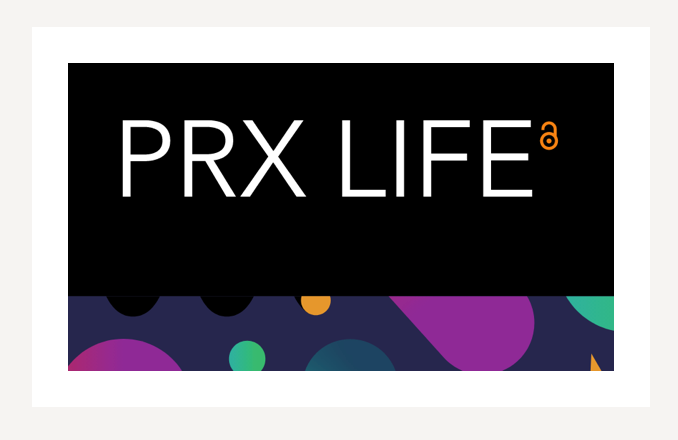
Authorization Required
Other options.
- Buy Article »
- Find an Institution with the Article »
Download & Share
Sign up to receive regular email alerts from Physical Review B
- Forgot your username/password?
- Create an account
Article Lookup
Paste a citation or doi, enter a citation.

IMAGES
VIDEO
COMMENTS
Critical exponent. Critical exponents describe the behavior of physical quantities near continuous phase transitions. It is believed, though not proven, that they are universal, i.e. they do not depend on the details of the physical system, but only on some of its general features. For instance, for ferromagnetic systems at thermal equilibrium ...
Experiments and simulations of the transition to turbulence in fluid flow through a quasi-2D channel reveal critical exponents consistent with directed percolation — long conjectured to be the ...
Determination of universal critical exponents using Lee-Yang theory. Lee-Yang zeros are points in the complex plane of an external control parameter at which the partition function vanishes for a many-body system of finite size. In the thermodynamic limit, the Lee-Yang zeros approach the critical value on the real axis, where a phase transition ...
These exponents describe the non-analyticity of various thermody-namic functions. Remarkably transitions as dierent as the liquid/gas and ferromagnetic transition can be described by the same set of critical exponents and are said to belong to the same Universality class. Those critical exponents most commonly encountered are defined below.
The remarkable thing about critical phenomena is that a bunch of different kinds of quantities - response functions like compressibility and others - follow this rule close to the critical temperature. There might be a different critical exponent for each different kind of quantity, but the same kind of expression represents the behavior of all of these quantities.
We find that the critical exponents of these fluids in this region are close to the exponents calculated from the three-dimen-sional Ising model. Theoretical insight into the critical behavior of fluids has been '' gained through a combination ' of phenomenology, model calculations, and, more ' recently, the renormalization- group approach.
We report precise optical measurements of the equations of state of Xe, S, and C very near their critical points (). We find that the critical exponents of these fluids in this region are close to the exponents calculated from the three-dimensional Ising model.
Through a combination of numerical and renormalization group arguments we resolve the contradiction between theory and experiment and demonstrate how the 'universal window' for critical exponents observed in experiment arises from a competition between marginal operators.
Consensus was reached at this conference that experimental asymptotic critical exponents of classical fluids and fluids mix-tures are in agreement with the exponent values for 3D Ising-like systems, since the cause of the apparent deviations of the series-expansions estimates from experiment [36, 37] and from theory [38, 40] had been found.
The various critical exponents are then defined as follows. The manner in which m 0 → 0, as T → T c from below, defines the exponent β: (1) m 0 ∼ ( T c − T) β ( h → 0, T ≲ T c). The manner in which the low-field susceptibility χ 0 diverges, as T → T c from above (or from below), defines the exponent γ (or γ ′ ): in the gas ...
Ising critical exponents This article lists the critical exponents of the ferromagnetic transition in the Ising model. In statistical physics, the Ising model is the simplest system exhibiting a continuous phase transition with a scalar order parameter and symmetry. The critical exponents of the transition are universal values and characterize the singular properties of physical quantities ...
A modest attempt to evaluate the critical exponents is made in Section 12.9, which yields results that are inconsistent with the experiment but teaches us quite a few lessons about the shortcomings of the so-called classical approaches.
The length-scaling exponent, μ, found experimentally sets constraints to the possible combinations of the critical exponents z and ν at a given interaction strength.
Through a combination of numerical and renormalization group arguments we resolve the contradiction between theory and experiment and demonstrate how the 'universal window' for critical exponents observed in experiment arises from a competition between marginal operators. Two-dimensional condensed matter is realized in increasingly diverse ...
Abstract We report precise optical measurements of the equations of state of Xe, SF_6, and CO2 very near their critical points (|T - T_c|/T_c < 5 x 10^-5). We find that the critical exponents of these fluids in this region are close to the exponents calculated from the three-dimensional Ising model. Citation Physical Review Letters Volume 37 ...
The critical exponent β calculated theoretically for the 3-dimensional Ising universality class is 0.326 ±0.002 [ 35 , 36 ]. Experimental values of the critical exponent β for a number of one ...
Abstract A review of theoretical predictions for the critical exponent in the Heisenberg model indicates that . Available measurements on Ni, all using the bulk technique, show a range of values between 1.28 and 1.35, with the best measurement falling at . In order to investigate this apparent discrepancy between theory and experiment, we have done a new measurement of via perturbed angular ...
The most important characteristics of a continuous phase transition are its critical exponents. The critical exponents are a quantitative characteristic of the critical fixed point. Agreement of theory with experiment implies that the critical fixed point studied theoretically is the same fixed point ob-served in the experiment.
As a consequence, the critical exponent κ = p /2 ν∼ 0.38, if we assume p = 2 is in good agreement with the κ- value obtained in our experiment.
Crystals. The experimentally-accessible critical regimes of the cuprate superconductors [1] excited a great interest in experiments on the normal-superconducting phase transition in these materials. Scaling theory predicts that the phase transition is governed by the static and dynamic critical exponents ν and z, respectively [2]. This theory ...
By measuring the transmitted light as a function of both temperature and wavelength, the critical exponent can be derived and the effects of light scattering with wavelength investigated. ", T1 - An undergraduate laboratory experiment to determine the critical point of SF6 using light scattering at selected wavelengths.
This length derived from the survival probability is compared with the distance from the transition point, yielding a critical exponent of at the phase boundary. Our experiment provides an alternative route to characterizing topological phase transitions and extracting their key physical quantities. Received 30 May 2022 Revised 5 August 2022
I love lord of the rings and i recently did a middle-earth lore dive so today we collectively cry about what modern hollywood did to rings of power season 2 ...
More broadly, Moscow has stepped up its barrage against the energy network, with Kyiv warning it faces the toughest winter in its history as a result of power shortages. "The most important priority is strengthening our air defense systems to protect Ukraine's energy infrastructure from enemy attacks," said Chernyshov.
We report an estimate [2.587,2.598] of the critical exponent of the Chalker-Coddington model of the integer quantum Hall effect that is significantly larger than previous numerical estimates and in disagreement with experiment. This suggests that models of noninteracting electrons cannot explain the critical phenomena of the integer quantum ...
The network is facing a crisis as a result of Russian strikes on critical infrastructure. In a missive released later Tuesday, Kudrytsky said he had called for a meeting of Ukraine's parliament to dispute the decision, saying the stated reasoning — that he had failed to maintain the network's security in the face of Moscow's drones and ...